Overview
Cosmochemistry is defined, and its relationship to geochemistry is explained. We describe the historical beginnings of cosmochemistry, and the lines of research that coalesced into the field of cosmochemistry are discussed. We then briefly introduce the tools of cosmochemistry and the datasets that have been produced by these tools. The relationships between cosmochemistry and geochemistry on the one hand and astronomy, astrophysics, and geology on the other are considered.
1.1 What Is Cosmochemistry?
A significant portion of the universe is comprised of elements, ions, and the compounds formed by their combinations – in effect, chemistry on the grandest scale possible. These chemical components can occur as gases or superheated plasmas, less commonly as solids, and very rarely as liquids.
Cosmochemistry is the study of the chemical compositions of the universe and its constituents, and the processes that produced those compositions. This is a tall order, to be sure. Understandably, cosmochemistry focuses primarily on the objects in our own solar system, because that is where we have direct access to the most chemical information. That part of cosmochemistry encompasses the compositions of the Sun, its retinue of planets and their satellites, the almost innumerable asteroids and comets, and the smaller samples (meteorites, interplanetary dust particles or “IDPs,” returned lunar samples) derived from them. From their chemistry, determined by laboratory measurements of samples or by various remote-sensing techniques, cosmochemists try to unravel the processes that formed or affected them and to fix the chronology of these events. Meteorites offer a unique window on the solar nebula – the disk-shaped cocoon of gas and dust that enveloped the early Sun some ~4.57 billion years ago, and from which planetesimals and planets accreted (Fig. 1.1).
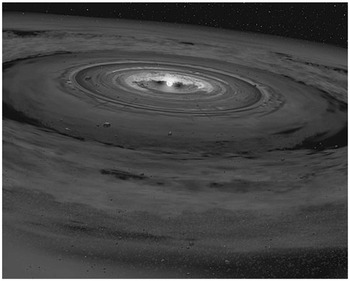
Figure 1.1 An artist’s conception of the solar nebula, surrounding the violent young Sun.
Within some meteorites are also found minuscule presolar grains, which provide us with an opportunity to analyze directly the chemistry of interstellar matter. Some of these tiny grains are pure samples of the matter ejected from dying stars, and they provide constraints on our understanding of how elements were forged inside stars before the Sun’s birth. Once formed, these grains were released into the interstellar medium (ISM), the space between the stars. The ISM is filled primarily with diffuse gases, mostly hydrogen and helium, but with oxygen, carbon, and nitrogen contributing about 1% by mass and all the other elements existing mostly as micrometer-sized dust motes. Much of the chemistry in the ISM occurs within relatively dense molecular clouds, where gas densities can reach 103–106 particles/cm3, high by interstellar standards (but not by our everyday experience: Earth’s atmosphere has ~3 × 1019 atoms/cm3 at sea level). These clouds are very cold, with temperatures ranging from 10 to 100 K, so interstellar grains become coated with ices. Reactions between ice mantles and gas molecules produce organic compounds that can be extracted from meteorites and identified by their bizarre isotopic compositions. Many dust grains were undoubtedly destroyed in the ISM, but some hardy survivors were incorporated into the nebula when the molecular cloud collapsed, and thence were accreted into meteorites.
Processes that occur inside stars, in interstellar space, and within the solar nebula have no counterparts in our terrestrial experience. They can be studied or inferred from astronomical observations and astrophysical theory, but cosmochemical analyses of materials actually formed or affected by these processes provide constraints and insights that remote sensing and theory cannot. Our terrestrial experience places us on firmer ground in deciphering the geologic processes occurring on the Earth’s Moon. In studying lunar rocks and soils, we can use familiar geochemical tools developed for understanding the Earth. We have also measured the chemical compositions of some other planetary bodies and their smaller cousins, geologically processed planetesimals, using telescopes and instruments on spacecraft. In some cases, we even have meteorites ejected during impacts onto these bodies. Chemical measurements (whether from laboratory analyses of samples or in situ analyses of rocks and soils by orbiting or landed spacecraft) add quantitative dimensions to our understanding of planetary science. All extraterrestrial materials are fair game for cosmochemistry.
1.2 Geochemistry versus Cosmochemistry
Traditionally, cosmochemistry has been treated as a branch of geochemistry – usually defined as the study of the chemical composition of the Earth. Geochemistry focuses on the chemical analysis of terrestrial materials, as implied by the prefix “geo,” and geochemistry textbooks commonly devote only a single chapter to cosmochemistry, if the subject is introduced at all. However, the line between geochemistry and cosmochemistry has always been somewhat fuzzy. The most prominent technical journal in this discipline, Geochimica et Cosmochimica Acta, has carried both names since its inception in 1950. The burgeoning field of planetary geochemistry appropriates the “geo” prefix, even though its subject is not Earth. A broader and more appropriate definition of geochemistry might be the study of element and isotope behavior during geologic processes, such as occur on and within the Earth and other planets, moons, and planetesimals. Using this definition, we will include planetary geochemistry as an essential part of our treatment of cosmochemistry.
It is worth noting, though, that the geochemical and cosmochemical behaviors of elements do show some significant differences. A geochemical perspective of the Periodic Table is illustrated in Figure 1.2 (adapted from Railsback, Reference Railsback2003). As depicted, this diagram is decidedly Earth-centric, but the controls on element behavior during geologic processes apply to other bodies as well. Determining relative elemental abundances is an important part of geochemistry, and the relative abundances of elements in the Earth’s crust vary over many orders of magnitude. Crustal abundances are illustrated in Figure 1.2, because most geochemical data are based on readily accessible samples of the crust. Geochemistry is also concerned with determining the composition of the Earth’s interior – its mantle and core – and a more comprehensive figure would include those abundances as well. Very few native elements (pure elements not chemically bound to any others) occur naturally in the Earth, so Figure 1.2 distinguishes elements that occur commonly as cations or anions (positively and negatively charged particles, respectively). The electrical properties of elements control how they combine into compounds (minerals), dissolve in natural fluids, or are concentrated into melts (magmas) at high temperatures. The elements in Figure 1.2 are also grouped by their so-called geochemical affinities: lithophile (rock-loving) elements tend to form silicates or oxides (the constituents of most rocks), siderophile (iron-loving) elements combine with iron into metal alloys, chalcophile (sulfur-loving) elements react with sulfur to form sulfides, and atmophile elements tend to form gases and reside in the atmosphere. Many elements exhibit several affinities, depending on conditions, so the assignments illustrated in Figure 1.2 offer only a rough approximation of the complexity of element geochemical behavior. Finally, an important part of geochemistry takes advantage of the fact that most elements have more than one isotope. Measuring isotopic abundances has great value as a geochemical tool, and the most commonly used isotope systems are illustrated by boxes with heavy lines in Figure 1.2. Stable isotopes of some light elements provide information on sources of elements, the conditions under which minerals form, and the processes that separate isotopes from each other. Unstable (radioactive) nuclides and their decay products (radiogenic nuclides) similarly constrain element sources and geologic processes, as well as permit the ages of rocks and events to be determined. The isotopic compositions of many other elements in terrestrial materials are now being analyzed, and a future Figure 1.2 will certainly expand the list of commonly used isotopic systems.
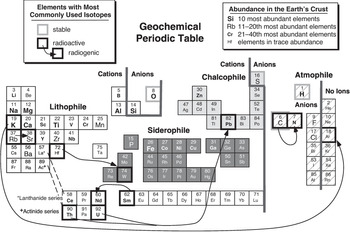
Figure 1.2 A geochemical Periodic Table, illustrating controls on element behavior during geologic processes. Relative abundances of elements in the Earth’s crust are indicated by symbol sizes. Cations and anions are usually combined into minerals. Elements having affinities for silicate or oxide minerals (lithophile), metal (siderophile), sulfide minerals (chalcophile), and gas (atmophile) phases are distinguished. Elements having stable isotopes that are commonly used in geochemistry are shown as boxes with bold gray outlines. Radioactive and radiogenic isotopes used for chronology are shown by boxes with bold black outlines and arrows showing decay relationships.
By way of contrast, Figure 1.3 illustrates a cosmochemical perspective of the Periodic Table. The element abundances shown in this figure are atomic concentrations in the Sun (relative to the abundance of silicon), as best we can determine them. The Sun comprises >99.8% of the mass of solar system matter, so solar composition is approximately equivalent to the average solar system (often incorrectly called cosmic) composition. The behavior of elements in space is governed largely by their volatility, which we quantify by specifying the temperature interval at which elements change state from a gas to a solid on cooling. (The liquid state is not generally encountered at the very low pressures of space; liquids tend to be more common in geochemistry than cosmochemistry.) All elements occur as gases at high enough temperatures, and they either condense at lower temperatures to form solid minerals or ices, or react with already condensed phases to form other solid phases. Some elements condense at such low temperatures that they effectively remain as gases. Thermodynamic data can be used to predict the temperatures at which solid phases become more stable than their components in a gas of solar composition. Assignment of elements to the various refractory and volatile groups in Figure 1.3 is based on the temperature at which 50% of each element has condensed into solid phases. It is convenient in cosmochemistry to identify elements according to the kinds of minerals into which they condense – lithophile, siderophile, and chalcophile. Some volatile elements only condense at very low temperatures to form ices, or do not condense at all. Also illustrated in Figure 1.3 are the most commonly used isotope systems in cosmochemistry; the complete list is considerably longer than for geochemistry, and would include stable isotopes measured in presolar grains in meteorites, cosmogenic nuclides formed by interaction with cosmic rays in space, and now-extinct radioactive isotopes that existed in the early solar system.
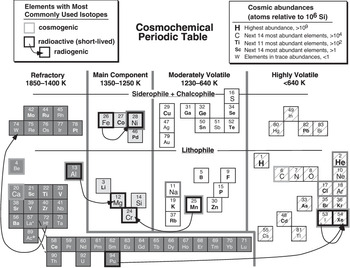
Figure 1.3 A cosmochemical Periodic Table, illustrating the behavior of elements in chondritic meteorites. Relative solar system abundances are indicated by symbol sizes. Volatilities of elements reflect the temperatures at which 50% of each element would condense into a solid phase from a gas of solar composition. As in Figure 1.2, the chemical affinities of each element – lithophile for silicates and oxides, siderophile for metals, and chalcophile for sulfides – are indicated. Some of the most highly volatile phases may have remained uncondensed in the nebula. Stable, radioactive, and radiogenic isotopes used in cosmochemistry are indicated by bold outlines, as in Figure 1.2. Abundances and 50% condensation temperatures come from tabulations by Lodders and Fegley (Reference Lodders and Fegley1998).
Comparison of Figures 1.2 and 1.3 reveals that the chemical behavior of an element may differ depending on whether it is in a geochemical or cosmochemical environment. This book’s topics will refer to both figures in understanding the compositions of extraterrestrial materials. In cosmochemistry we are concerned with the origin and behavior of elements in space, whereas in planetary geochemistry we focus on their behavior once they are accreted into bodies that undergo geologic (usually thermal) processing. Planetary geochemistry follows more or less the same rules as on the Earth, although these rules must be modified to accommodate different geologic conditions or starting compositions. And the geochemical consequences of biology, so important on Earth, do not apply on other worlds, so far as we can determine presently.
1.3 Beginnings of Cosmochemistry (and Geochemistry)
1.3.1 Philosophical Foundations
The philosophical foundations of cosmochemistry date to the last half of the eighteenth century when Immanuel Kant (1724–1804) and Pierre-Simon Marquis de Laplace (1749–1827) put forward comprehensive models for the origin of the solar system. Kant’s model, published in 1755, started with the Sun at the center of a gaseous nebula. In order for this cocoon of gas and dust to be stable in the gravitational field of the Sun, the nebula had to rotate about the Sun. Kant suggested that the matter in the disk would segregate into large bodies that would become the planets. This segregation would take place slowly, with each body developing into a miniature version of the solar system. Kant showed that the rotation of the planets and their satellites would be in the same sense as their revolution around the Sun. In 1796, Laplace published a model that started with the primordial Sun occupying the entire volume now occupied by the planetary orbits. This hot, luminous “solar nebula” rotated as a rigid body so that linear velocity was greatest at the outer edge. As the nebula cooled and contracted, it rotated faster to preserve angular momentum. When centrifugal force exceeded gravitational attraction, a ring was left behind. This process was repeated many times and the rings contracted to form planets. During the nineteenth century, these two models became intertwined into a “nebular hypothesis” that was generally accepted in some form until the beginning of the twentieth century. Ideas based on these models, such as a hot solar nebula, have remained part of mainstream cosmochemical thought until very recently.
1.3.2 Meteorites and Microscopy
Meteorites (Fig. 1.4) are central to cosmochemistry, because they are our most accessible source of extraterrestrial samples. Though people have seen stones falling from the sky for thousands of years, the fact that meteorites actually fall was not acknowledged by the European and American scientific establishments until early in the nineteenth century. Credit for putting meteorites on the scientific map generally goes to Ernst Chladni (1756–1827). In a 63-page book with the long title (translated from German) On the Origin of the Mass of Iron Found by Pallas and of Other Similar Iron Masses, and on a Few Natural Phenomena Connected Therewith, published in 1794, Chladni laid out a case based on historical records of observed falls that stone and iron masses enter the Earth’s atmosphere from space and form fireballs as they plunge through the atmosphere. These ideas contradicted two beliefs that were strongly held by his scientific contemporaries: rocks and masses of metal do not fall from the sky, and no small bodies exist in space beyond the Moon. However, during the next five years, four falls of stony meteorites were witnessed and widely reported in Europe. Chemist Edward Howard (1774–1816) and mineralogist Jacques-Louis de Bournon (1751–1825) carried out a series of chemical and mineralogical analyses of stones said to have fallen from the sky and found that they were similar in texture and composition, and significantly different from terrestrial rocks. The publication of these findings in early 1802 was followed by the fall in 1803 of nearly 3000 stones at L’Aigle in Normandy, France. These events provided evidence to support Chladni’s claims, and meteorites entered the realm of scientific study.
A major step in understanding meteorites came with Henry Clifton Sorby’s (1826–1908) development of the petrographic microscope in the mid-1800s. Using this instrument, thin sections (paper-thin slices of rock, mounted on glass slides) are observed by passing polarized light through them from below, providing a means of identifying minerals and observing the textures of rocks. Sorby soon turned his attention to a type of meteorite called chondrites, describing the round droplets of solidified melt in them (called chondrules, after the Greek “chondros” for “grains” or “seeds”) as drops of a fiery rain (Fig. 1.5). Chondrites will be described in detail in Chapter 6.
A significant part of meteoritics literature focuses on petrographic description and classification. This does not usually make for exciting reading, but an orderly classification is essential for interpreting the chemical compositions of meteorites and recognizing relationships among them. Beginning in the 1860s, Gustav Rose (1798–1873) at the University Museum of Berlin and Nevil Story-Maskelyne (1823–1911) at the British Museum developed meteorite taxonomies based on microscope observations. Gustav Tschermak (1836–1927) later refined Rose’s classification, and Aristides Brezina (1848–1909) refined the Rose–Tschermak classification, which reached its final form in 1904. This classification was based on mineralogy, because at the time there were few chemical analyses of meteorites, and those that existed were of uneven quality. George Prior (1862–1936) devised a simpler mineralogical classification for chondrites in 1920. Prior’s major mineralogical subdivisions for meteorites are still used today, but his system has been supplanted by one devised by Randall Van Schmus and John Wood (Reference Van Schmus and Wood1967) that separates primary characteristics of chondrites, such as bulk composition, from secondary characteristics, such as degree of metamorphic or aqueous alteration. We will discuss meteorite classification in detail in Chapter 6.
1.3.3 Spectroscopy and the Compositions of Stars
In the early nineteenth century, determining the compositions of the Sun and other stars posed a fundamental hurdle for astronomy. The French philosopher Auguste Compte (1798–1857) confidently asserted that never, by any means, would we be able to study the chemical compositions of celestial bodies. But spectroscopy soon proved him wrong. Spectroscopes attached to telescopes were used to spread out starlight into its component wavelengths. The spectra of stars and of the Sun showed numerous narrow, dark gaps where particular wavelengths were missing. These gaps (absorption lines) are due to the various chemical elements in a star’s outer layers absorbing light emanating from the hotter interior. Each element absorbs (or emits) light at specific wavelengths characteristic of its electronic structure.
Joseph Lockyer (1836–1920) was one of the pioneers of solar spectroscopy. In examining the spectra of solar prominences in 1869, Lockyer noticed an absorption line that he could not identify. Reasoning that it represented an element not present on Earth, he proposed a new element – helium, from the Greek word “helios” for “Sun.” This idea failed to achieve acceptance by Lockyer’s scientific colleagues, until a gas having the same mysterious spectral line was found 25 years later in rocks. The helium in terrestrial uranium ore formed as a decay product of radioactive uranium. Thus, this abundant element was first discovered in the Sun rather than in the laboratory. Lockyer’s cosmochemical discovery was recognized by the British government, which created a solar physics laboratory for him. Lockyer also founded the scientific journal Nature, which he edited for 50 years.
In the late 1800s, after decades of work on the spectroscopy of stars, Lockyer developed his “meteoritic hypothesis.” According to this idea, meteorites were the primary dust of the universe. Nebulae observed by astronomers were interpreted as swarms of meteorites bound together through gravitation and interacting much like atoms in a gas. Lockyer postulated that the solar system and other objects had formed from these meteorite swarms (Lockyer, 1890). Although the original hypothesis was soon abandoned, the idea that meteorites might be chemically primitive materials that sample the cosmos was not far off the mark.
Identifying the elements present in the Sun and stars from their spectra was one thing, but determining their relative abundances was quite another. The solar absorption lines for iron are particularly prominent, leading astronomers to believe that iron was the most abundant element in the Sun, as it is in the Earth and in many meteorites. Princeton astronomer Henry Russell (1877–1957) even conjectured that if the Earth’s crust were heated to the temperature of the Sun, its spectrum would resemble the solar spectrum. It took until the 1920s before a clear understanding of how spectra arise was established, permitting evaluation of the true compositions of the Sun and stars. The key to understanding stellar spectra was discovered in 1925 by Cecilia Payne (1900–1979). She showed that the spectral lines arose from the excitation of the electrons surrounding the atomic nucleus and that the energy levels of the electrons were a function of stellar temperature. When temperature was taken into account, the abundances of elements in stars were shown to be nearly the same in a variety of stars, in spite of them having different spectra. Her work also showed that hydrogen and helium are the most abundant elements in the Sun and other stars. This last result was not widely immediately accepted and was downplayed in her published thesis. But by 1930, her work had completely supplanted previous interpretations and modern spectroscopy was born.
1.3.4 Solar System Element Abundances
The term “cosmochemistry” apparently derives from the work of Victor Goldschmidt (Fig. 1.6), who is often described as the father of geochemistry. This is yet another crossover and, in truth, Goldschmidt also established cosmochemistry as a discipline. In 1938, he published a cosmic abundance table based on the proportions of elements in meteorites. He used the term “cosmic” because, like his contemporaries, he believed that meteorites were interstellar matter. Chemist William Harkins (1873–1951) had formulated an earlier (1917) table of elemental abundances – arguably the first cosmochemistry paper, although he did not use that term. As explained in Chapter 4, solar system abundance is now preferred over cosmic abundance, although the terms are often used interchangeably.

Figure 1.6 Victor Goldschmidt, as pictured on a Norwegian postage stamp issued in 1974.
Goldschmidt and his colleagues in Germany, and later in Norway (where he escaped the grasp of the Nazis in World War II), analyzed and compiled a wealth of chemical data on terrestrial rocks and meteorites. The compositions of terrestrial rocks have been modified by partial melting (leaving some components behind as residues) and by fractional crystallization (where crystals are segregated from the melt, causing the liquid’s composition to change). However, Goldschmidt recognized that chondrites have not experienced wholesale melting and have thereby escaped geologic processing. They are basically cosmic sediments – physical mixtures of nebular matter whose chemical abundances have remained unchanged since they formed. To obtain accurate compositions using the then-new analytical technique of emission spectroscopy, Goldschmidt separated chondrites into their more readily measurable silicate, metal, and sulfide components and analyzed each in turn. Consequently, he was able to determine how various elements were partitioned among these coexisting phases (thereby inventing the terms “lithophile,” “siderophile,” and “chalcophile” to describe their geochemical affinities). He then calculated what he called the “cosmic abundances” of 66 elements by using the weighted means of element concentrations in meteorite silicate (10 parts), metal (2 parts), and sulfide (1 part). At about the same time, astronomers began using the Sun’s spectra to estimate elemental abundances. It soon became apparent that solar elemental abundances were similar to Goldschmidt’s cosmic (chondritic) abundances, except that the meteorites were depleted in the most volatile elements like hydrogen and helium. Cosmic abundances (more appropriately called solar system abundances) are a cornerstone of cosmochemistry because they represent the raw material from which the solar system formed.
1.3.5 Isotopes and Nuclear Physics
Isotopes were recognized at the beginning of the twentieth century as a result of studies of radioactivity. Careful studies found three naturally occurring radioactive decay series – the thorium, uranium, and actinium series – all of which ended with stable lead. The existence of isotopes was confirmed in 1911 when Fredrick Soddy (1877–1956) measured the atomic masses of lead obtained from uranium-rich and thorium-rich ores and showed that they were different. During this same period, J. J. Thompson (1856–1940) discovered that ions accelerated through an electric field would adopt different parabolic trajectories depending upon their masses. Thompson’s student, Francis Aston (1877–1945), used this principle to design several different mass spectrographs, which separated particles by mass/charge ratio and recorded the output on photographic plates. By 1920, Aston was reporting the presence and relative abundances of isotopes of numerous elements, including oxygen, neon, argon, krypton, xenon, and mercury (Aston, 1920). In 1922, he received the Nobel Prize in Chemistry for his work on isotopes using a mass spectrograph. However, an understanding of the structure of the atom had to await the discovery of the neutron in 1930.
During the 1930s, further technological advances permitted detailed studies of the masses and relative abundances of isotopes. Modern mass spectrometers designed by Alfred Nier (1911–1994) at the University of Minnesota had greater mass-resolving power and were more sensitive than any built previously. Nier made accurate measurements of the isotopic abundances of argon, potassium, zinc, rubidium, and cadmium and, in the process, discovered 40K, which would later become an important isotope for dating rocks. He also pioneered the development of uranium–lead and thorium–lead dating.
In 1940, Nier successfully separated 235U from 238U using a mass spectrometer, providing an enabling technology for the Manhattan Project. During World War II, many of the top nuclear physicists worked on the development of the atomic bomb. The fascinating story of these years and their effect upon the participants is beyond the scope of this book. But as the war ended, many of them turned their attention away from the tools of war toward understanding our planet and universe, and their knowledge became available to cosmochemistry.
The leading figure in cosmochemistry during the 1950s and 1960s was Harold Urey (1893–1981). Urey (Fig. 1.7) was one of the first practitioners of cosmochemistry as we understand it today. He was awarded the Nobel Prize in Chemistry in 1934 for his work on deuterium and heavy water. During the war, he and his colleagues developed the gaseous diffusion method for separating 235U from 238U. After the war, he became a professor at the University of Chicago, where he did pioneering work using the 18O/16O ratio in paleoclimate research, developed theories about the origin of the elements and their abundances in stars, pointed out the importance of short-lived radionuclides such as 26Al, investigated the origins of life on Earth, and made many other contributions. He was a leader in developing the scientific rationale for returning samples from the Moon.
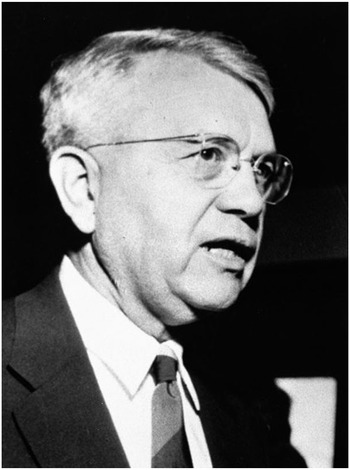
Figure 1.7 Harold Urey, one of the fathers of cosmochemistry.
During World War II, Hans Suess (1909–1993) was part of a team of German scientists working on heavy water. In 1950, he emigrated to the United States. His work with Urey on nucleosynthesis and the abundances of the elements is a cornerstone of cosmochemistry. In 1965, along with Heinrich Wänke (1928–2015), he proposed that the extremely high noble gas contents in some chondritic meteorites were due to the implantation of solar wind. He also worked on climate research and 14C dating. Together, Suess and Urey (Reference Suess and Urey1956) published the first table of cosmic abundances to include the abundances of the isotopes.
The new knowledge of nuclear physics affected cosmochemistry in another way. A classic paper by astrophysicists Margaret and Geoffrey Burbidge (husband and wife), William Fowler, and Fred Hoyle (this paper was so influential that it has come to be known by scientists simply as “B2FH”), and a similar contribution by Alastair Cameron, both published in 1957, provided the theoretical basis for understanding how elements are produced in stars, as described in Chapter 3.
Radiometric dating using long-lived radionuclides came into its own in the 1940s and 1950s with the advent of better mass spectrometers. The uranium-isotope decay scheme was first shown to be useful as a geochronometer by Fritz Houtermans and Arthur Holmes in 1946. The first accurate determination of the age of the Earth was made in 1956 by Clair Patterson, who used the uranium–lead method to date meteorites. The 40K–40Ar decay scheme was shown to be a useful chronometer for meteorites by Gerald Wasserburg in his doctoral thesis, completed in 1954. The first age determination by the 87Rb–87Sr method was published by Hahn et al. (1943), and the method came into wide use in the 1950s. Its application to meteorites peaked in the late 1960s and 1970s, in conjunction with work on the lunar samples.
The first short-lived radionuclide (one whose primordial abundance has decayed away) was shown to have been present in meteorites by John Reynolds in 1960. Reynolds found large excesses of 129Xe, the decay product of short-lived 129I, in chondritic meteorites. This discovery showed that elements had been synthesized in stars shortly before the formation of the solar system. A more important short-lived radionuclide, 26Al, was demonstrated to have been present in meteorites by Typhoon Lee et al. in 1977. This isotope is particularly significant, as it is thought to have been a potent source of heating for asteroids and planets early in solar system history. A variety of other short-lived isotopes have now been confirmed in meteorites and are the basis for high-resolution chronometry of the early solar system.
Nuclides formed by nuclear reactions induced by high-energy cosmic rays are called cosmogenic. Cosmogenic isotopes are more common in meteorites than on the Earth, because our planet’s atmosphere screens out most cosmic rays. However, meteorites traveling in space are heavily irradiated by cosmic rays, and the production of cosmogenic isotopes can be used to estimate the times since the meteorites were liberated from their parent asteroids (these times are called cosmic-ray exposure ages). The first cosmic-ray exposure ages were measured in the late 1950s. Since then, thousands of cosmic-ray exposure ages have been estimated using a variety of cosmogenic nuclides, and new modeling techniques have allowed the interpretation of complex irradiation histories.
In 1956, John Reynolds pioneered a new and highly sensitive method for measuring noble gases, which effectively created the field of noble gas geochemistry and cosmochemistry. Noble gases have many isotopes and, because they do not bond with rock-forming elements, they have very low abundances in most materials. Thus, additions from the decay of radioactive nuclides or cosmic-ray interactions are easy to detect. Noble gases also exhibit different elemental and isotopic ratios in meteorites, reflecting different processes operating in the early solar system. In addition, isotopic anomalies that could not be explained by any processes known to be operating in the solar system were found in xenon (discovered by John Reynolds and Grenville Turner in Reference Reynolds and Turner1964) and neon (discovered by David Black and Robert Pepin in Reference Black and Pepin1969) extracted from meteorites. These noble gases provided the first hints that presolar grains might have survived in the nebula, although they were not widely recognized at the time.
The pursuit of the carriers of exotic noble gas components by Edward Anders and colleagues at the University of Chicago and in other laboratories eventually led to the isolation of presolar grains from meteorites. The approach used by Anders involved laborious tracking of exotic noble gas carriers through steps of increasingly harsh chemical dissolutions and physical separations. He was rewarded with the discovery of presolar diamond (the first isolated presolar grain), silicon carbide, and graphite, the carriers of the three main exotic noble gas components. These three materials are all carbon rich, but subsequent work has identified presolar oxides, nitrides, and silicates, as discussed in Chapter 5.
1.3.6 Spacecraft, Returned Samples, and Remote Chemical Analyses
The launch of Sputnik by the Soviet Union in 1957 changed the world forever. The immediate impact was to change the nature of the Cold War by demonstrating the feasibility of intercontinental ballistic missiles. But Sputnik also raised the curtain on the scientific exploration of space and on visiting and obtaining samples from other solar system bodies. The first target was the Moon. Close-up images of the Moon were provided by the United States’ Ranger missions, which impacted the Moon in 1964 and 1965. The first lunar chemical data were provided by the Soviet Luna and American Surveyor spacecraft in 1966. The first manned landing on the Moon was the Apollo 11 mission in 1969. Five more successful Apollo missions followed, before the program was abruptly terminated.
The return of 381 kg of lunar rocks and soils from six sites on the Moon’s nearside by Apollo astronauts (Fig. 1.8) and 326 g from three sites by Soviet Luna robotic landers in the 1970s (Table 1.1) provided a bonanza of new extraterrestrial materials for cosmochemistry. The intense interest in these samples encouraged a considerable expansion of laboratory techniques and capabilities. Fortuitously, two large meteorites (the Allende and Murchison chondrites) fell to Earth in 1969, just as the new laboratories were gearing up for lunar sample return. The new analytical techniques were applied to these meteorites, initially as a means of demonstrating capability, but the two chondrites turned out to be incredibly interesting in their own right and provided a new impetus for the study of all types of extraterrestrial materials. Because lunar samples were so precious, many groups simultaneously analyzed the same rocks, and competition forced the quality of the analyses to new heights. Lunar rocks were especially useful because mapping of the Moon by telescopes and orbiting spacecraft provided the geologic context for these samples. Lunar soils allowed for wider sampling of rock types, because the soils consist of rock particles thrown tremendous distances by impacts. In addition, lunar soils contain implanted solar wind particles, providing a window onto solar element abundances. A decade after the Apollo program ended, the first lunar meteorites were recognized in Antarctica. Their discovery, made possible by comparing them with Apollo samples, proved that rocks could be ejected from one body and travel to another. For the first time, rocks delivered by natural processes from another planet were available for direct geochemical analysis. While the Apollo and Luna samples were collected from a geographically restricted area (<5% of the surface area) of the Moon, the nearly 150 distinct lunar meteorites (as of time of writing) are thought to provide a more representative sample. In 2020, China’s Chang’e 5 spacecraft returned ~2 kg of lunar samples from a young volcanic mound, the only returned lunar samples in the last four decades (Table 1.1).
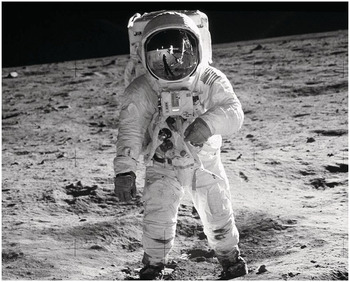
Figure 1.8 Apollo astronaut on the Moon.
Table 1.1 Samples returned by spacecraft missions
Sample source | Mass | Date Returned to Earth | Mission |
---|---|---|---|
Moon: Mare Tranquilitatis | 21.55 kg | Jul 24, 1969 | Apollo 11 (NASA) |
Moon: Oceanus Procellarum | 34.30 kg | Nov 24, 1969 | Apollo 12 (NASA) |
Moon: Mare Fecunditatis | 101 g | Sep 24, 1970 | Luna 16 (USSR) |
Moon: Fra Mauro Highlands | 42.80 kg | Feb 9, 1971 | Apollo 14 (NASA) |
Moon: Hadley–Apennine | 76.70 kg | Aug 7, 1971 | Apollo 15 (NASA) |
Moon: Apollonius Highlands | 55 g | Feb 25, 1972 | Luna 20 (USSR) |
Moon: Descartes Highlands | 95.20 kg | Apr 27, 1972 | Apollo 16 (NASA) |
Moon: Taurus–Littrow | 110.40 kg | Dec 19, 1972 | Apollo 17 (NASA) |
Moon: Mare Crisium | 170.1 g | Aug 22, 1976 | Luna 24 (USSR) |
Earth–Sun Lagrange 1 | Solar wind atoms | Sep 8, 2004 | Genesis (NASA) |
Comet Wild 2 | 1000s of dust particles | Jan 15, 2006 | Stardust (NASA) |
Asteroid 25143 Itokawa | 1000s of particles | Jun 13, 2010 | Hayabusa (JAXA) |
Asteroid 162173 Ryugu | ~5.4 g | Dec 5, 2020 | Hayabusa2 (JAXA) |
Moon: South Pole–Aitken | ~2 kg | Dec 2020 | Chang’e 5 (CNSA) |
Asteroid 101955 Bennu | ~250 g? | Sep 2023 (planned) | OSIRIS-REx (NASA) |
Remote sensing of the Moon by spacecraft complemented the return of samples. Using X-ray fluorescence spectrometers, the Apollo 15 and 16 service modules obtained orbital measurements of aluminum, silicon, and magnesium at a spatial resolution of ~50 km. In 1994, the Clementine orbiter mapped the surface reflectance of the Moon at 11 wavelengths with spatial resolutions of 100–300 m. These multispectral images have been used to infer mineralogy as well as the abundances of iron and titanium. In 1997–1998, the Lunar Prospector orbiter used a gamma-ray spectrometer (GRS) to obtain global maps of the abundances of iron and thorium at 15 km spatial resolution and potassium and titanium at 60 km resolution. Additional GRS data for oxygen, magnesium, aluminum, silicon, calcium, and uranium were obtained at 150 km resolution, and a neutron spectrometer measured hydrogen concentrations in lunar soils. In 2009, the Lunar Reconnaissance Orbiter and its LCROSS impactor characterized the lunar radiation environment and found water ice at one of the poles. In 2011, the GRAIL mission, consisting of two orbiters, refined our understanding of lunar gravity, and LADEE studied the atmosphere and airborne dust in 2013. Besides the United States and Russia, other spacefaring nations (the European Union, Japan, China, and India) have sent probes to the Moon that have made remote-sensing measurements.
The first close-up images of the planet Mars were returned by the Mariner 4 spacecraft, which flew by the planet in 1964. Mariners 6 and 7 flew past Mars in 1969, returning more images, and Mariner 8 was the first spacecraft to go into orbit around Mars in 1971. The Viking missions landed two spacecraft on Mars in 1976. The Viking landers took high-resolution images of their landing sites and gathered data on the composition of the atmosphere and surface soils.
In 1979, two unusual meteorites, both igneous rocks, were hypothesized to be Mars samples. This controversial idea was based primarily on their young crystallization ages (several hundred million years), because it was difficult to envision how melting could occur on small asteroids so late in solar system history. The idea found wide acceptance several years later when trapped gases in these meteorites were found to have chemical compositions identical to the martian atmosphere, as measured by the Viking landers (Fig. 1.9). Three decades later, nearly 150 separate martian meteorites have been recognized. The chemical compositions of these meteorites – shergottites, nakhlites, chassignites, commonly abbreviated to SNCs – have provided a wealth of new insights into geochemical processes on Mars.
More recently, chemical analyses of martian rocks and soils were obtained using α-particle X-ray spectrometers (APXS) on the Mars Pathfinder rover (Sojourner) in 1997, two long-lived Mars Exploration Rovers (Spirit and Opportunity) beginning in 2004, and the Mars Science Laboratory rover (Curiosity) beginning in 2012 (Fig. 1.10). The mineralogy of martian surface materials has been determined by a thermal emission spectrometer (TES) on the Mars Global Surveyor orbiting spacecraft at spatial resolutions of a few tens of kilometers, and higher spatial resolution mineralogical mapping has been provided by a thermal emission mapping spectrometer on Mars Odyssey and visible/near-infrared spectrometers on Mars Express (OMEGA) and the Mars Reconnaissance Orbiter (CRISM). Abundances and global distributions of iron, potassium, thorium, silicon, calcium, and chlorine have also been determined by a GRS on the Mars Odyssey orbiter. A neutron spectrometer determined concentrations of hydrogen in the martian subsurface. In 2005, Phoenix landed near the northern martian pole and probed for ice. The Curiosity rover, carrying a variety of chemical and mineralogical tools, has explored an ancient martian lake bed since 2012. MAVEN and the Trace Gas Orbiter are analyzing the martian atmosphere, and the InSight lander studied Mars’ interior structure beginning in 2018. The Mars 2020 rover (Perseverance) was launched in 2020 and will explore Jezero crater beginning in 2021. This rover will analyze samples using X-ray fluorescence (PIXL) and ultraviolet native fluorescence and Raman spectra (SHERLOCK), as well as cache samples for possible future return to Earth. The United Arab Emirates and China have also launched spacecraft intending to perform remote-sensing measurements on Mars.

Figure 1.10 A family portrait of Mars rovers. Sojourner (Mars Pathfinder rover) is in the foreground, Spirit (Mars Exploration Rover, MER) is on the back left, and Curiosity (Mars Science Laboratory, MSL) is on the right. Perseverance (Mars 2020 rover, not pictured) is approximately the size of Curiosity.
Several Soviet Venera and Vega spacecraft landed on the surface of Venus in the early 1980s and survived for a few minutes before succumbing to the stifling heat and pressure. X-ray fluorescence chemical analyses for a number of major elements in surface samples were reported. Chemical and isotopic analyses of the Venus atmosphere were made by Pioneer Venus, Venera, and Venus Express. In 1990, Magellan provided global radar maps of the surface.
The Mariner 10 spacecraft flew by Mercury and photographed one side of the planet in 1975. Mercury was not visited again until the MESSENGER spacecraft flew past the planet in 2008 and 2009 before achieving orbit in 2011. In addition to high-resolution imagery of the entire planet, MESSENGER acquired chemical and mineralogical information about the planet, studied its core and magnetic field, and investigated the composition of its very thin atmosphere.
Aspects of the chemical composition of the atmospheres of Jupiter, Saturn, Uranus, and Neptune were measured by the Voyager and Galileo spacecraft in the 1980s and 1990s, respectively. The Juno orbiter arrived at Jupiter in 2016 and is now measuring its composition. The atmospheres of Saturn and of Titan, Saturn’s largest satellite, were analyzed by the Cassini spacecraft and its Huygens probe (Fig. 1.11). Much of what is known or inferred about the compositions of satellites of the giant plants is based on the spectra of sunlight reflected from their cloud tops.
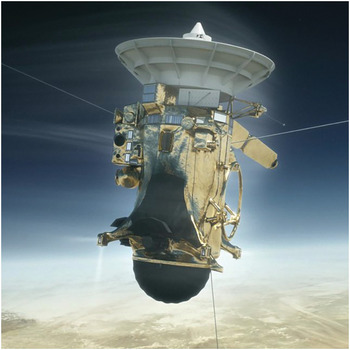
Figure 1.11 The nuclear-powered Cassini spacecraft, launched towards Saturn in 1997, was active for nearly 20 years. The ring on the right side held the Huygens probe, which parachuted onto Titan’s surface.
Several spacecraft have obtained in situ chemical data for asteroids. The NEAR Shoemaker spacecraft, which orbited asteroid Eros in 2000–2001, made compositional measurements of the surface using X-ray fluorescence (XRF) and gamma-ray spectra. The Japanese Hayabusa spacecraft, which visited asteroid Itokawa in 2003, also carried an XRF spectrometer and measured the surface composition. Both asteroids were found to have chondritic compositions. The Dawn spacecraft orbited asteroid Vesta in 2011, characterizing its mineralogy with visible/near-infrared spectra and its chemistry with a gamma-ray and neutron spectrometer. Vesta, the second most massive body in the asteroid belt, is thought to be the source for howardite, eucrite, and diogenite (HED) meteorites, and that idea was confirmed by Dawn. The spacecraft then traveled to the dwarf planet (formerly asteroid) Ceres, where it orbited and analyzed its surface composition and geology from 2015 to 2018 and discovered that it is a partly differentiated icy body.
The Hayabusa mission returned thousands of tiny particles from Itokawa to Earth (Table 1.1), which confirmed its classification as chondritic. Hayabusa2 rendezvoused with asteroid Ryugu in 2018. The spacecraft collected two samples totaling 5.4 g and returned them to Earth in 2020. OSIRIS-REx arrived in orbit around asteroid Bennu in 2018; it collected abundant surface samples in 2020 and will return them to Earth in 2023 (Table 1.1).
Spacecraft missions have visited five comets and have provided some spectacular images of the comet nuclei and data on their chemical and mineralogical compositions. In 1985, NASA’s ICE spacecraft passed through the tail of comet Giacobini–Zinner. In 1986, five spacecraft visited comet 1P/Halley (Halley’s Comet). The Soviet Vega 1 and Vega 2 probes sent images of the nucleus, and the European Giotto probe made a close pass by the nucleus. All three of these probes had mass spectrometers that returned data on the composition of dust expelled from the comet. Two Japanese probes also visited comet 1P/Halley. Comet Grigg–Skjellerup was imaged by the Giotto spacecraft in 1989. NASA’s demonstration spacecraft Deep Space 1 imaged the nucleus of comet Borrelly in 2001.
More ambitious comet missions have flown subsequently. The Deep Impact mission went to comet Temple 1, arriving in 2005. Deep Impact released a large impactor that crashed into the comet, and then studied the material that was released using spectrometers and cameras. Information on the chemical composition and mineralogy of the comet showed that it contains both low-temperature and high-temperature materials. The Stardust mission visited comet Wild 2 in 2004. In addition to taking some spectacular images, the spacecraft brought samples of comet dust back to Earth in 2006 (Table 1.1). Rosetta orbited comet Churyumov–Gerasimenko in 2014, conducting the most detailed comet observations to date. Its Philae lander detected organic compounds on the comet’s surface.
In 2016, New Horizons flew by Pluto, now demoted from planetary status to that of dwarf planet, and its moon Charon. New Horizons returned images and compositional analyses. Pluto is the innermost object of the Kuiper belt, which contains thousands of icy worlds that orbit beyond the outermost planet, Neptune. Continuing its traverse outward, the spacecraft flew by and imaged Arrokoth, a small Kuiper belt object, in 2019.
1.3.7 Multiple Sources of Extraterrestrial Materials
For many years, cosmochemistry depended on the chance discovery of meteorites – either witnessed “falls” and serendipitous “finds,” or the dogged determination of a few private collectors who systematically searched for them. That changed in 1969, when Japanese explorers in Antarctica led by Masaru Yoshida stumbled onto meteorites exposed on bare ice. American geologist William Cassidy immediately recognized an opportunity, and with support from the National Science Foundation he mounted a joint expedition with the Japanese to the Allan Hills region of Antarctica in 1977 to recover meteorites. This was the first of many expeditions, sponsored by the National Science Foundation and headed first by Cassidy and later by Ralph Harvey, that have returned to Antarctica every year to collect meteorites (Fig. 1.12). The Japanese have operated a parallel field program in the Yamato Mountains region of Antarctica. Other countries, including the countries that comprise the European Space Agency, China, and Russia have also sent meteorite-collecting expeditions to Antarctica. Altogether, these expeditions have recovered well over 55,000 meteorites (including paired specimens), which have dramatically increased the number of meteorites available to science. Among the meteorites returned by these expeditions were the first lunar meteorites and several other previously unknown meteorite types.
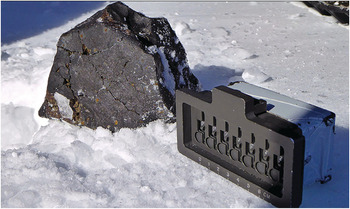
Figure 1.12 A frozen meteorite exposed on Antarctic ice. The numbers at the bottom of the identifying counter at the right are a centimeter scale.
Meteorites fall onto the Antarctic continent and become frozen into the ice. Glaciers, carrying this treasure trove of meteorites, constantly creep downslope towards the edges of the continent. Wherever a moving glacier is obstructed by a mountain, its flow stagnates and layers of ice are removed by wind ablation, exposing meteorites in great concentrations. American expeditions collect the meteorites and send them to NASA’s Johnson Space Center in Houston, where they are cut into pieces and allocated to scientists all over the world. Japanese expeditions return their samples to the National Institute for Polar Research in Tokyo for curation and allocation.
About the same time that meteorites were found in Antarctica, an important collection of meteorites was being put together in Roosevelt County, New Mexico. From 1966 to 1972, several meteorite hunters collected ~140 meteorite specimens, representing about 100 separate fall events. This collection demonstrated another way in which nature concentrates meteorites. The meteorites in Roosevelt County were found in “blowout” areas, where up to a meter of soil had been blown away by the wind, leaving meteorites in plain view on the hardpan surface. Based on this experience, systematic and successful searches of desert areas in Western Australia and the southwestern United States have been carried out. Subsequently, the deserts of North Africa have turned out to be especially prolific sources of meteorites. The shifting desert sands expose meteorites that have accumulated over thousands of years. The meteorites are collected by nomads and sold to Western collectors. Although most desert meteorites are weathered to some degree, new and rare meteorite classes have been discovered this way.
In 1974, astronomer Donald Brownlee began collecting IDPs in the stratosphere using collectors on the leading edges of airfoils on high-altitude aircraft. The smallest or fluffiest of these tiny particles (e.g. Fig. 1.13), those with the highest surface-to-volume ratio, are decelerated in the upper atmosphere without being substantially heated. The drifting particles can be either captured in the stratosphere or recovered from low-dust environments on the Earth’s surface for laboratory studies. Similar particles and slightly larger micrometeorites have also been collected frozen in polar ice. IDPs appear to be materials from asteroids and comets.
The Genesis spacecraft collected solar wind particles during two years in orbit. The solar collectors were returned to Earth in 2005, but unfortunately a parachute failed to open and the sample container ruptured during its hard landing. Although the collector devices were damaged by the impact and there was significant contamination, valuable data on the Sun’s composition have been, and continue to be, obtained from the damaged collectors.
In 2006, the Stardust spacecraft returned samples of comet Wild 2 to Earth. These samples are tiny particles expelled from the comet nucleus and captured in aerogel (a highly porous medium that can snag speeding cometary grains) when the spacecraft flew through the coma at high speed. Even though the deceleration caused the particles to disaggregate and portions of them to melt, some mineral grains were preserved intact, and elemental and isotopic signatures have been measured along the tracks in the aerogel and in particles at the ends of the tracks.
1.3.8 Organic Matter and Extraterrestrial Life?
Some meteorites, known as carbonaceous chondrites, contain several percent of carbon-bearing compounds, primarily organic molecules. The earliest characterizations of organic matter in chondritic meteorites were attempted in the late 1800s. Researchers speculated that the organic material was probably extraterrestrial in origin. However, interest soon waned and, curiously, not a single paper appeared on meteoritic organic matter between 1899 and 1953. However, interest in extraterrestrial organic compounds intensified in the 1960s, when Bartholomew Nagy and his colleagues published a series of papers claiming that the Orgueil carbonaceous chondrite contained biogenic hydrocarbons and “organized elements” that resembled fossilized algae. However, it was soon shown that the organic material came from terrestrial contamination, a problem that continues to plague organic cosmochemistry. The large amounts of meteoritic material available from the falls of the Murchison chondrite in 1969 and the Tagish Lake chondrite in 2000 generated considerable activity in determining both the structures and relative abundances of organic compounds and their stable-isotope compositions. Subsequent work has provided important new constraints on the processes that formed organic compounds in the ISM, the solar nebula, and on meteorite parent bodies.
Since ancient times, philosophers have wondered whether life on Earth was seeded from elsewhere in the universe, an idea known as “panspermia.” It is generally agreed that meteorites and comets contain prebiotic compounds that could have provided the raw material for life on Earth. A more contentious question is whether the comets might actually contain microscopic organisms, as proposed by Hoyle and Wickramasinghe (1981). This hypothesis has not been taken seriously by most scientists, although it is hard to disprove.
In Reference McKay, Gibson and Thomas-Keprta1996, David McKay et al. published a paper that claimed to show evidence for possible extraterrestrial life in the martian meteorite ALH 84001 (Fig. 1.14). One of the lines of evidence involved organic compounds found in this meteorite. The paper generated tremendous interest and controversy and resulted in the creation of a new NASA research initiative to study astrobiology. Intense study has provided nonbiological explanations for all the original claims. Nonetheless, the intense astrobiology research effort generated by the 1996 study is still going strong.
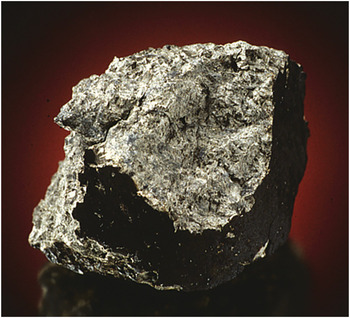
Figure 1.14 A piece of the ALH 84001 martian meteorite, ~8 cm across. Dark material on the surface is fusion crust, formed during passage through the Earth’s atmosphere. This sample created a stir when it was proposed to contain evidence for extraterrestrial life.
1.4 The Tools of Cosmochemistry
In this section, we briefly review the tools used by cosmochemists and the kinds of datasets that have been acquired over the years. The tools identified below are described in more detail in the Appendix at the end of this book. Drawing on the data gained from these tools and on the intellectual framework of cosmochemistry, we can formulate questions and define research protocols to answer key questions.
1.4.1 Laboratory and Spacecraft Analyses
1.4.1.1 Mineral Identification and Petrography
The most basic information about any object is obtained by examining it with our own senses. What does it look like? How heavy is it? Is it solid or crumbly? A powerful extension of these senses is the petrographic microscope, which is used to examine slices of rocks and meteorites that are thin enough to transmit light. A skilled petrographer can identify minerals based on their interactions with polarized light. Meteorite classification is heavily based on the mineralogical and textural characteristics of the samples. Petrography has also played a major role in inferring the geologic history of the Moon from lunar samples and of Mars from martian meteorites. Electron-beam instruments (electron microprobe, scanning electron microscope, transmission electron microscope) extend the petrographer’s senses by providing information on mineralogy and crystal structure at a much higher spatial resolution.
Minerals can also be identified by remote sensing, using spectroscopy. Reflectance spectra have absorption bands in the visible/near-infrared region caused by electronic transitions, commonly of iron in certain crystal sites. Thermal emission spectra are produced when solar energy is absorbed by minerals and re-emitted at thermal infrared wavelengths. Both types of spectra can be diagnostic for specific minerals, and spectroscopic measurements are made using telescopes and instruments on orbiting or landed spacecraft. A recent flight instrument is the ChemCam instrument on the Curiosity rover on Mars. A laser beam vaporizes grains in a target rock, and spectrographs identify elements in the plasma. Specific minerals can sometimes be identified if the analyzed spot is only one phase. A Raman spectrometer is included on the Perseverance Mars rover. Raman and luminescence spectroscopes are being developed for future Mars rover missions.
X-ray diffraction (XRD), a mainstay in mineralogical identification on Earth, has been applied on Mars by the CheMin instrument on the Curiosity rover. This tool has provided the most definitive identification of minerals that are present in low abundance.
1.4.1.2 Mineral Chemistry
Prior to the 1960s, mineral compositions were determined either by wet chemical analyses of mineral separates or by reference to an extensive database of the optical properties of minerals as functions of their compositions. With the development of the electron microprobe, which uses electrons to excite characteristic X-rays, it became possible to measure directly the chemical composition of each mineral in a thin section. The energy-dispersive X-ray detector available on most scanning electron microscopes can provide in situ chemical analyses of minerals. Certain mineral compositions can also be determined from spectroscopy.
1.4.1.3 Bulk-Rock Chemistry (Major and Minor Elements)
During the nineteenth century and the first half of the twentieth century, bulk chemical compositions of meteorites were determined by wet chemistry techniques. The most reliable bulk chemical datasets were compiled by Hugo Wiik and Eugene Jarosewich. During the second half of the twentieth century, new methods were established to determine chemical compositions. XRF analysis was used extensively during the 1950s and 1960s. In this technique, a beam of X-rays excites electrons in the sample, which then lose this energy by re-emitting X-rays that are unique to each element. XRF has also been used to analyze a few elements on the lunar surface from orbit, and was used by the Viking landers to carry out more complete analyses of soils on Mars in 1975.
During the 1960s, this technique was largely supplanted by neutron activation analysis. In this technique, small samples are placed into a nuclear reactor, and neutrons from the reactor interact with the elements in the sample to produce radioactive isotopes. The sample is then removed from the reactor and the radioactive isotopes decay, emitting characteristic gamma rays, which are then counted to determine the chemical composition of the sample. Neutron activation analysis can detect 74 elements, but unfortunately silicon, a dominant element in most cosmochemical samples, cannot be directly measured. Today, datasets based on this technique provide a cornerstone for ideas about meteorites and lunar samples.
Gamma-ray and neutron spectrometers on orbiting spacecraft have been used to determine elemental abundances in surface samples from the Moon, Mars, and several asteroids. Gamma rays are produced by nuclear transitions, such as those that occur during interactions of surface materials with solar cosmic rays. The energy of gamma rays identifies specific nuclei, which can be translated into abundances for a handful of elements. Accurate major and minor element abundances in Mars rocks and soils have also been determined by APXS on four rovers. Alpha particles (4He nuclei) produced by radioactive decay bombard a sample, producing X-rays that are characteristic of specific elements. A microfocusing XRF spectrometer (PIXL) is part of the instrument package on the most recent Mars rover, Perseverance.
1.4.1.4 Trace-Element Abundances
The distributions of trace elements between minerals and within a suite of related rocks provide powerful tools for constraining the origin and history of rocks and meteorites. Trace-element abundances for rocks are typically part of the dataset collected when determining bulk compositions. Trace-element compositions of minerals require more powerful techniques such as the ion microprobe (secondary ion mass spectrometer, or SIMS) or laser-ablation inductively coupled plasma mass spectrometry (LA-ICPMS).
Potassium and thorium, which normally occur in minor or trace abundances, have been determined in surface materials on the Moon, Mars, and an asteroid by gamma-ray spectroscopy from orbiting spacecraft.
1.4.1.5 Radiogenic Isotopes and Chronology
Radiometric dating utilizes the constant decay rates of radioactive (unstable) isotopes to determine how much time has passed since an object formed. Long-lived radionuclides (those for which some of the primordial abundance is still present in the solar system) provide absolute ages for objects relative to today. Short-lived, now-extinct radionuclides provide high-resolution relative ages for objects that formed in the earliest epochs of solar system history. Mass spectrometry is the main tool for determining ages by radiometric dating.
Radiometric dating is not the only method for determining age relationships. Relative ages can be determined by examining the textural relationships between various objects. For example, an object enclosed by another object must have formed earlier. Some complex relative chronologies can be developed based on textural observations. A melt that contains a mineral grain unrelated to the melt must have formed after the mineral grain that it contains. On a larger scale, a rock unit that stratigraphically overlies or crosscuts another rock formation must have formed after the underlying formation. The relative age of a planetary surface can also be estimated from the density of impact craters on that surface. Cratering rates can be calibrated by radiometric dating of rocks from that surface or by comparison with other surfaces that have been dated. Using all these techniques, detailed chronologies can and have been developed for solar system materials.
Cosmic-ray exposure dating is used to determine how long a meteorite has traveled through space as a small object on the way to Earth from its parent asteroid, or how long a material was exposed to cosmic rays on the surface of a planet (or the Moon) during its history. Exposure to high-energy cosmic rays alters the isotopic chemistry of the target material, and measurement of the products of these interactions can be used to determine cosmic-ray exposure ages.
1.4.1.6 Stable-Isotope Compositions
Chemical and physical processes affect the stable isotopes of an element differently because of slight variations in the energy levels of the electrons caused by different numbers of neutrons in the nucleus of each isotope. These slight differences result in mass-dependent fractionations (changes in relative abundances of the isotopes) that are characteristic of these processes. For example, during precipitation of a mineral from a fluid, the isotopic composition of the mineral will differ slightly from that of the fluid. The magnitude of the difference is typically temperature dependent, so the compositions of the liquid and the mineral can be used to define the temperature at which the mineral formed. This is an example of mass fractionation during an equilibrium process. As another example, if a melt evaporates into empty space, the isotopic composition of the melt becomes heavier as lighter isotopes are preferentially lost. The isotopic composition of the residual melt will be proportional to the amount of evaporation. This is an example of kinetic isotope fractionation. As with radiometric dating, the primary tool for this type of analysis is the mass spectrometer.
The Curiosity rover carried the Sample Analysis at Mars (SAM) instrument – actually a suite of instruments: mass spectrometer, gas chromatograph, and tunable laser spectrometer – that measured the abundance of light elements and their stable-isotope compositions.
1.4.1.7 Organic Compounds
Organic molecules are identified by a variety of techniques, after they have been extracted from chondrites using liquid solvents or stepwise heating. Mass spectrometers can separate many compounds and identify them by their masses. The SAM instrument on the Curiosity rover has identified organic molecules on Mars. Other techniques can identify reactive functional groups or specific structural types of molecules. A visible/near-infrared spectrometer on the Dawn spacecraft used this approach to identify organic molecules on Ceres.
1.4.2 Cosmochemical Theory
A powerful theoretical tool for cosmochemical models is thermodynamics. This formalism considers a system in a state of equilibrium, one consequence of which is that observable properties of the system undergo no net change with time. (We offer a somewhat more rigorous discussion of thermodynamics in Chapter 7.) The tools of thermodynamics are not useful for asking questions about a system’s evolutionary history. However, with the appropriate equations, we are able to estimate what a system at equilibrium would look like under any environmental conditions. The methods of thermodynamics allow for the use of temperature and pressure, plus the system’s bulk composition, to predict which minerals will be stable and in what relative amounts they will be present. In this way, the thermodynamic approach to a cosmochemical system can help us measure its stability and predict the direction in which it will change if environmental parameters change.
Cosmochemistry literature often contains discussions of equilibrium condensation from a gas of solar composition. During the 1960s through the 1980s, the intellectual framework for these discussions was a hot, monotonically cooling solar nebula (recall the Laplace model mentioned earlier). However, the discovery in chondrites of isotopic anomalies that could not have survived in the gaseous state and grains that existed in interstellar space prior to the formation of the solar system showed that this simple model for the early solar system was not strictly correct. Cosmochemists still refer to condensates, and the previous work still provides important constraints for understanding solar system bodies. However, the application of these principles is generally in reference to a local environment or specific situation rather than a global environment like a hot solar nebula. We are also now more aware that thermodynamics reveals nothing about the pathways to the final states that we observe, and that similar compositions can result from condensation or evaporation.
In the planetary geochemical literature, there also are extensive discussions about the thermodynamics of crystallizing melts. Sophisticated programs have been developed to model the compositions of the minerals that crystallize from a cooling liquid, as well as changes in the residual melt.
A third way in which thermodynamics can be used is to invert the measured major, minor, and trace-element compositions of coexisting minerals to estimate the temperature, pressure, and oxidation state under which they formed. Valid results can only be obtained if the system was truly in equilibrium at the inferred conditions and the minerals did not subsequently change composition. Nevertheless, this is a powerful tool to infer the formation conditions of various solar system materials.
An alternative way to study a system is to examine its kinetics. Using kinetics, we can study the pathways along which a system may evolve between states of thermodynamic equilibrium and determine the rates of change of system properties along those pathways. Cosmochemical systems can evolve along a variety of pathways, some of which are more efficient than others. In a kinetic study, the task is often to determine which of the competing pathways is dominant.
Cosmochemists are interested in determining not only what should have happened in a real system (the thermodynamic answer) but also how it is most likely to have happened (the kinetic answer). The two are intimately linked. While the focus of thermodynamics is on the end states of a system – before and after a change has taken place – a kinetic treatment sheds light on what happens between the end states. Where possible, it is advantageous to keep both approaches in mind.
Theories of the origin of the solar system and of the formation and evolution of the bodies within it provide the intellectual framework for detailed investigations of processes and events in the early solar system. These theories are often based primarily on work in fields other than cosmochemistry as we have defined it. For example, observations made by astronomers tell us about the environments in which stars form. Astrophysics modelers describe the gravitational collapse of dense cores in molecular clouds to form protostars and accretion disks. Dynamicists model the evolution of planetary systems starting with the solar nebula and continuing through the formation of the planets and the evolution of the distribution of asteroids and icy bodies as we see them today. Input from these and other disciplines generates global models that provide the often-unstated framework for cosmochemistry. Cosmochemists must be familiar with these broad ideas, but must also test them against observations from their own research. Observations are the ultimate arbiter of a successful theory.
1.5 Relationship of Cosmochemistry to Other Disciplines
It should be clear from the above discussions that cosmochemistry is a highly interdisciplinary subject that draws on aspects of chemistry, physics, geology, astronomy, astrophysics, and perhaps eventually even biology. Understanding the formation of the elements requires consideration of the astrophysical settings in which nucleosynthesis occurs – stars, supernovae, and the ISM. Astronomy provides direct observations of these settings, as well as spectroscopic data on chemical abundances that complement chemical analyses of presolar grains in meteorites. Astrophysics provides the theoretical predictions of relative abundances of nuclides produced in various stellar settings that can be tested by the chemical and isotopic compositions of presolar grains. Hypotheses about the formation of organic molecules in interstellar space can be evaluated by studying organic matter in meteorites. Spectroscopic elemental abundances measured in the Sun can be compared with element ratios in bulk chondritic meteorites, and the agreement allows measured chondritic abundances to be substituted for those elements without measurable solar spectral lines. Astrophysical models of changes in the composition of the Sun over time can be tested by analyzing solar wind gases implanted into soils on the Moon and asteroids. Nowhere in science is there a better example of synergy between disparate disciplines.
Likewise, planetary geochemistry cannot be interpreted without understanding its geologic context. Geologic processes on Mars, the Moon, and other planets can be inferred from spacecraft imagery, and the conditions under which they occur can be determined by in situ measurements by orbiters or landers. Element behavior during melting and crystallization can be inferred by analyzing martian and lunar meteorites and returned lunar samples. The timing of major geologic events and the ages of mantle reservoirs can be defined by radiogenic isotope measurements. Geochemistry and geology provide complementary ways of assessing how planets work.
Small bodies such as asteroids and comets contain primordial material but may also have experienced heating and impacts. Metamorphism (mineralogical and textural changes wrought by heat and shock) can blur or even destroy the cosmochemical and chronological records in meteorites, and it is essential to catalog these geologic processes so that we can interpret the records correctly. Melting and sequestering of siderophile elements in some asteroid cores produced iron meteorites, analyses of which shed light on an inaccessible region of our own planet. Astronomical observations and spectroscopy of asteroids complement direct measurements of their chemical compositions by analyses of meteorites.
In this book, we will explore cosmochemistry and planetary geochemistry, usually in the context of astronomy, geology, and physics. In this way we hope to illuminate how the abundances and chemical behaviors of elements and isotopes are critical to understanding the formation and inner workings of the Earth’s neighboring planets, the processes that shaped the early solar system, the chronology of major events, and the origins of the elemental building blocks of the universe.
Questions
1. How does cosmochemistry relate to other, more well-known disciplines such as geology, chemistry, and astronomy?
2. Distinguish between the following terms: lithophile, siderophile, chalcophile, atmophile.
3. How do element behaviors in the solar nebula or in interstellar space differ from those in planets?
4. Who was Victor Goldschmidt and what role did he play in the development of geochemistry and cosmochemistry?
5. What are some of the sample sets that are available to cosmochemists?
6. List five techniques by which elements and/or isotopes can be measured.