Introduction
The proportion of the global population aged 60 years and over is expected to increase over the coming decades. In a recent report by the UN, the number of adults aged 60 years or more in the USA is expected to rise from 74·6 million in 2015 to 122·7 million by 2050(1). Multiple studies have indicated that older adults demonstrate markedly reduced cerebral structural integrity than younger adults, which in turn predicts poorer cognitive functioning(2–5). As such, the identification of factors associated with cerebral structural integrity is paramount. Moreover, if these factors are modifiable, they may be targeted by therapeutic interventions to support cerebral structural integrity and therefore cognitive function with age.
There is a growing literature indicating that nutritional intake may be a pertinent factor influencing the structural integrity of the cerebrum. In the present review, we initially discuss how cerebral structure changes with age but also how such change relates to cognitive functioning. Following this, we discuss the extent to which dietary intake (inferred by FFQ or blood biomarker concentrations) of B vitamins (predominately B6, B12, and folate/folic acid), choline, n-3 fatty acids (n-3-FA) or vitamin D predicts cerebral structural integrity in dementia-free older adults. We will also discuss whole diets such as Mediterranean- (MeDI) and Western-style diets (WeDI), and whether adherence to these dietary patterns predicts cerebral structure. Although the majority of studies within the current literature, and therefore the present review, are cross-sectional or prospective by design, we also discuss the limited number of randomised clinical trials (RCT) that have been performed in this field. Overall, we suggest that nutritional intake is a key lifestyle factor that may have the potential to modify the trajectory of cerebral structural integrity with age.
Cerebral structural integrity in older adults without dementia
MRI has been widely used to investigate cerebral structural integrity changes with age, as well as how such change relates to cognition. The most common measures of cerebral structural integrity include whole or regional cerebral volumes (total volume or compartmentalised grey and white matter (WM) volumes), cortical thickness (CT; the thickness of the cerebral cortex; globally and regionally) as well as the presence and volume of WM hyperintensities (WMH). WMH are bright, or hyper-intense, foci on fluid attenuated inversion recovery (FLAIR) MRI scans, typically due to extensive myelin and axonal deterioration(6,7).
While each of the aforementioned methods are useful for examining large-scale changes to cerebral structure (cerebral macrostructure), they are poorly suited for examining cerebral structure at the microscopic level (cerebral microstructure). For investigating the latter, an MRI methodology termed diffusion tensor imaging (DTI) is frequently utilised. The most common DTI metrics describing cerebral microstructural integrity are fractional anisotropy (FA) and mean diffusivity (MD). Higher FA and lower MD values are suggestive of more intact cerebral microstructure(8). However, FA and MD are summary measures and provide little detail regarding the factors underpinning microstructural decay. To this end, the DTI metrics radial diffusivity (RD) and axial diffusivity (AxD) are often examined. Data derived from rodent studies have revealed that axonal damage appears to facilitate reduced AxD(9–11) whereas demyelination appears to trigger increased RD(12,13). In the present review, poorer cerebral structural integrity refers to relative reductions in whole/regional cerebral volumes, increased frequency or volume of WMH, or poorer global, regional or tract specific microstructural integrity as measured by FA, MD, RD or AxD using DTI.
Cerebral macrostructural integrity in cognitively normal adults
Cross-sectional and longitudinal studies have observed a robust trend towards reduced total cerebral volume (TCv) with increasing age(14–19), with the annual decline in TCv estimated to be between 0·28 and 0·55%(16,20,21). Senescence-related structural decline is evident throughout each cerebral lobe in cognitively normal adults(18,22–25) though frontal and parietal lobes appear to exhibit the greatest decline with increasing age(18,22).
Contributing to changes in TCv are declines to both total and regional grey matter (GM) volumes. A range of studies report that GM volume demonstrates linear decline with increasing age(5,14–16,22,26–29), whereas others report non-linear deterioration indicative of accelerated atrophy typically after midlife(30,31). Overall, age-related reductions in GM volume appear to be relatively widespread with reduced volume having been identified within frontal regions including the superior, middle and inferior frontal gyri as well as orbito-frontal cortex; temporal regions such as the inferior, middle and superior temporal gyri in addition to the temporoparietal junction; superior and inferior parietal lobes; and various occipital zones(5,17,25,26,29,30,32–42). Likewise, medial temporal volumes (i.e. entorhinal cortex, hippocampus and parahippocampus) demonstrate decline with increasing age in cognitively normal older adults(14,19,21,22,24,25,30,37,41,43–45).
Similar to GM, cerebral WM volume is reduced in older adults relative to younger adults. However, unlike GM where atrophy may be evident from young adulthood onwards(35), reductions in WM volume are typically apparent from midlife (4th to 6th decade), before which volume typically increases(16,22,23,31,45–47). In addition, the frequency and volume of WMH located within deep (periventricular) and subcortical WM increases with age(4,19,48–51), especially within the frontal and temporal lobes(4).
Cerebral microstructural integrity in cognitively normal older adults
Cerebral microstructural integrity also demonstrates decline with increasing age. Compared with younger adults, cognitively normal adults demonstrate reduced FA and increased MD(2,3,47,52–63), as well as increased RD(2,47,54,55,58,60,62) throughout cerebral WM. Conversely, regional increases and decreases in AxD have been observed in cognitively normal older adults relative to their younger counterparts(55). Poorer cerebral microstructural integrity in older adults (reduced FA, increased MD, or increased RD), compared with young adults, is evident within association (cingulum, fornix, superior/inferior longitudinal and orbito-frontal fasciculi, uncinate and perpendicular fasciculi), commissural (corpus callosum) and projection fibre tracts (corona radiata, and internal capsule)(2,57,58,61,62,64–70). These findings indicate that senescence-related microstructural decline within the brain of cognitively normal adults is quite pervasive.
Cerebral structural integrity in older adults with age-associated memory impairment or mild cognitive impairment
While there are many data demonstrating reduced cerebral structural integrity in cognitively normal adults with increasing age, other work has identified a greater magnitude of decline in adults with conditions such as age-associated memory impairment (AAMI) or mild cognitive impairment (MCI). Compared with cognitively normal adults, older adults diagnosed with MCI typically demonstrate greater reductions in TCv(32,71,72) as well as increased atrophy within frontal, parietal, temporal, and medial temporal lobes(73–82). A previous systematic review has similarly demonstrated smaller hippocampal volumes in older adults with AAMI compared with their cognitively normal counterparts(73).
Likewise, degradation of cerebral WM appears to be greater in adults with MCI than those deemed cognitively normal. Across older adults, diagnosed as cognitively normal or as having MCI or Alzheimer’s disease, Smith et al. (83) observed a trend of increasing WMH volume with clinical progression of cognitive dysfunction (cognitively normal < MCI < Alzheimer’s disease). Similar observations have been made by a number of other groups comparing cognitively normal older adults and those with MCI(78,84–86).
There also appears to be an increased magnitude of cerebral microstructural deterioration in adults diagnosed with MCI. Multiple studies have observed comparatively lower FA, as well as increased MD and RD in adults with MCI compared with those with normal cognitive functioning(87–99). Further, increased MD, RD and AxD as well as reduced FA scores are apparent within the WM of the fornix and temporal stem of older adults with MCI compared with those with AAMI(100).
Cerebral structure and cognitive performance in older adults without dementia
In a number of studies, poorer cerebral structural integrity appears to predict poorer cognitive performance. In cognitively normal adults, or those with MCI, lower total/regional GM volumes and reduced CT have correlated with reduced processing speed, as well as poorer global functioning, memory performance and executive functioning(4,5,27,42,78,79,82,101–104). Within these same groups, reductions in WM volume as well as an increased number of WMH predicted reduced processing speed as well as poorer global functioning, executive function, problem solving, memory performance and learning ability(7,49,51,83,84,101,105–108).
The quality of cerebral microstructure also appears to be a strong predictor of cognitive performance in dementia-free older adults. Poorer cerebral microstructure, indicated by decreased FA or increased MD or RD, has been correlated with poorer processing speed, executive functioning, problem solving, cognitive flexibility, inhibition and reasoning, pattern recognition, verbal fluency, as well as learning and memory(2,3,49,64–68,88,91,92,95,98,99,109–123).
Summary
Poorer cerebral structural integrity is apparent with increasing age. However, change does not appear unitary, with adults with conditions such as AAMI or MCI typically demonstrating greater cerebral structural decline than cognitively normal adults. Importantly, in each of these cohorts, poorer cerebral structural integrity appears to be correlated with reduced cognitive performance across multiple domains.
Nutritional intake and cerebral structural integrity in older adults without dementia
Although cerebral structural integrity declines with increasing age, there is also increasing evidence that persistently held lifestyle factors may influence the rate of decline. One such factor appears to be nutritional intake. While the current literature is limited, and of varying quality, the nutrients that have been investigated as potential predictors of cerebral structural integrity include B vitamins (particularly B6, B12 and folate), choline, n-3-FA and vitamin D. Adherence to prudent (for example, MeDI) or unhealthy diets (for example, WeDI) has also been investigated as a possible predictor of cerebral structure, as has the consumption of certain whole foods (for example, fish or alcohol). There is even greater scarcity of RCT examining the effects of nutritional supplementation on cerebral structural integrity. To date, only supplementation with B vitamins (i.e. B6, B12 and folate), n-3-FA or polyphenols (specifically resveratrol) has been investigated as a means of modifying cerebral structural integrity in dementia-free older adults.
The following is an overview of cross-sectional and longitudinal studies examining the relationship between the intake of key nutrients (inferred by FFQ or blood biomarker concentrations) and cerebral structural integrity in older adults. While some studies do not report whether their samples included dementia patients, only those involving dementia-free samples (or mixed groups which either adjusted for dementia diagnosis or repeated analysis following their exclusion) are summarised in online Supplementary Table S1. In addition to the overview of cross-sectional and longitudinal studies, an exhaustive review of available RCT investigating nutritional supplementation and cerebral structure in dementia-free adults is provided below. These specific RCT are summarised in Table 1.
Table 1 Randomised clinical trials examining cerebral structure following chronic nutritional supplementation in older adults without dementia (presented in reverse chronological order and by nutrient type)
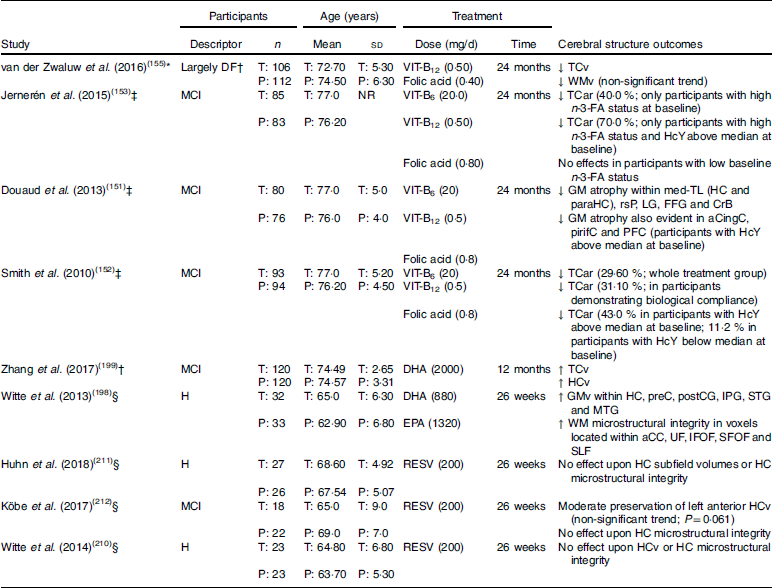
DF, dementia free; T, treatment group: P, placebo group; VIT-B12, vitamin B12; ↓, lower/reduced; TCv, total cerebral volume; WMv, white matter volume; MCI, mild cognitive impairment; NR, not reported; VIT-B6, vitamin B6; TCar, total cerebral atrophy rate; n-3-FA, n-3 fatty acid; HcY, homocysteine; GM, grey matter; med-TL, medial temporal lobe; HC, hippocampus; paraHC, parahippocampus; rsP, retrosplenial precuneus; LG, lingual gyrus; FFG, fusiform gyrus; CrB, cerebellum; aCingC, anterior cingulate cortex; pirifC, piriform cortex; PFC, prefrontal cortex; ↑, higher/increased; HCv, hippocampal volume; H, healthy; GMv, grey matter volume; preC, precuneus; postCC, postcentral gyrus; IPG, inferior parietal gyrus; STG, superior temporal gyrus; MTG, middle temporal gyrus; WM, white matter; aCC; anterior corpus callosum; UF, uncinate fasciculus; IFOF, inferior fronto-occipital fasciculus; SFOF, superior fronto-occipital fasciculus; SLF, superior longitudinal fasciculus; RESV, resveratrol.
* Structural MRI only performed post-intervention.
† Participants only screened with the Mini-Mental State Examination, of which only six scored <25 (3 % of sample; treatment group range, 19–30; placebo group range, 24–30; no significant difference between groups).
‡ Structural MRI performed pre- and post-intervention.
§ Structural MRI and diffusion tensor imaging performed pre- and post-intervention.
B vitamins
B group vitamins, specifically vitamins B6, B12 and folate, are readily available from a number of different whole foods. Vitamin B6 is available from meat (including organ meats), but also eggs, nuts, soya beans, and vegetables such as potatoes. The best food sources for vitamin B12 appear to be meat or meat products (for example, milk, cheese or eggs) whereas folate may be obtained through the consumption of foods such as dark green vegetables (for example, broccoli and spinach), as well as asparagus and liver(124). The aforementioned B vitamins are also obtainable through the consumption of dietary supplements, fortified cereals and beverages.
Similar to other nutrients, estimating the mean dietary intake of B vitamins is possible through the accurate administration and scoring of an FFQ or nutrition diary. The extent to which B vitamins are ingested in the diet may also be reflected by specific blood biomarkers. Aside from concentrations of these vitamins in plasma, blood biomarkers used to infer B vitamin intake (specifically B6, B12 and folate) include holotranscobalamin (holoTC), transcobalamin (TC), methylmalonic acid (MMA) and homocysteine (HcY). Higher concentrations of holoTC and TC are indicative of greater ingestion of vitamin B12. Conversely, higher concentrations of MMA may reflect a poorer intake of vitamin B12, while elevated HcY may reflect deficient or insufficient intake of vitamin B6, B12 or folate(125–127).
In an early cross-sectional study, Erickson et al. (128) determined mean intake of vitamins B6 and B12 in a sample of healthy community-dwelling older adults using a 3 d nutrition diary. In their analysis, greater estimated intake of vitamin B6 was correlated with increased GM volume of the anterior and posterior cingulate cortices, medial parietal cortex, left parietal lobe, supplementary motor area, as well as bilateral superior frontal and middle temporal gyri. Conversely, increased estimated intake of vitamin B12 only correlated with increased GM volume within the posterior parietal lobe. Interestingly, the associations with vitamins B6 and B12 were only evident when examining estimated intake from diet and dietary supplementation, not diet alone. This would suggest that intake from supplementation was responsible for these effects.
More recently, others have examined cerebral macrostructural integrity in relation to B vitamin blood biomarkers. In a study of 107 healthy older adults, aged 61–87 years, Vogiatzoglou et al. (126) observed that low concentrations of plasma vitamin B12, holoTC and TC predicted greater global cerebral atrophy, though no relationship was observed for MMA or HcY levels following covariate adjustment. Conversely, Tangney et al. (129) observed that higher serum HcY and MMA levels correlated with lower TCv. Similarly, de Lau et al. (130) has also observed greater WMH volume co-occurring with lower concentrations of plasma B12, HoloTC, and TC, as well as increased MMA. More recently, Köbe et al. (131) examined hippocampal microstructural integrity in adults with MCI with different levels of serum vitamin B12. Köbe et al. (131) identified lower microstructural integrity (increased MD) within the dentate gyrus (DG) as well as cornu ammonis 4 (CA4) in adults with low-normal serum vitamin B12 (<304 pmol/l) compared with adults with higher concentrations (≥304 pmol/l). The association between vitamin B12 and the microstructure of DG and CA4 also partially mediated the relationship between vitamin B12 and memory performance.
Blood HcY concentration appears to be a particularly robust predictor of cerebral structural integrity in older adults. Greater ventricular volume, lower regional GM volumes, and elevated rates of cortical or total cerebral atrophy are evident in older adults with higher HcY(132–137). Likewise, Rajagopolan et al. (138) identified a negative association between frontal, parietal and occipital lobe WM volumes and HcY, even after controlling for participant cognitive function (cognitively normal, MCI or Alzheimer’s disease). Specifically, for every 5 µmol/l increase in HcY, TCv was reduced by 4·4%. However, these effects were restricted to adults with MCI during subgroup analysis. More recently, Madsen et al. (139) examined the correlation between plasma HcY, CT, cortical GM volume and cortical surface area in a large sample of older adults with varying levels of cognitive function (normal cognitive function, MCI or Alzheimer’s disease). Although no relationship was observed between HcY and cerebral structure when considering each group separately, when all participant data were collated (controlling for age, sex and diagnosis), higher HcY predicted reduced CT as well as cortical GM volume, but not surface area, in multiple regions within the frontal, parietal and temporal lobes. Similarly, others have identified a negative correlation between HcY and hippocampal volume in dementia-free older adults aged 60 to 91 years(133,140,141). Higher HcY concentrations have also been correlated with an elevated rate of WM atrophy, as well as an increased frequency and severity of WMH and silent brain infarcts(129,140,142–148). Several other studies using DTI have also observed elevated HcY predicting poorer cerebral microstructure(149,150).
Several RCT have also investigated whether chronic supplementation with B vitamins (B6, B12, and folic acid) influences cerebral structural integrity in older adults. In the Homocysteine and B vitamins in cognitive impairment (VITACOG) trial, adults diagnosed with MCI were administered an oral B vitamin supplement (B6 20 mg/d; B12 0·5 mg/d; folic acid 0·8 mg/d) for 2 years. Several secondary analyses of VITACOG trial data identified that B vitamin supplementation supported cerebral structural integrity through reducing the rate of total cerebral atrophy, as well as GM atrophy within the frontal, temporal and medial temporal lobes(151,152). These effects appear to be greatest in adults demonstrating biological compliance (increased vitamin B12 or folate status), who also exhibited higher baseline HcY levels.
In a more recent analysis of VITACOG trial data, Jernerén et al. (153) likewise observed that B vitamin treatment appeared to reduce cerebral atrophy rate in adults diagnosed with MCI. However, these effects varied depending on baseline n-3-FA status and HcY concentrations. Whilst no significant treatment effect on cerebral atrophy was evident in adults with low baseline n-3-FA status or HcY concentrations (<11·3 µmol/l), B vitamin treatment appeared to reduce cerebral atrophy rate by 40% in adults with high n-3-FA status. n-3-FA status also appeared to modify the cerebral effects following B vitamin treatment in adults with high baseline HcY concentrations (≥11·3 µmol/l). Specifically, cerebral atrophy rate was reduced by approximately 70% following B vitamin treatment in adults demonstrating elevated HcY concentrations at baseline in addition to high n-3-FA status, whilst no effect was apparent in those adults with low n-3-FA status. Similar observations were made by Oulhaj et al. (154) using VITACOG trial data, though for cognitive performance.
A second RCT examining cerebral structural integrity in response to chronic B vitamin supplementation is the MRI substudy of the B-vitamins for the Prevention Of Osteoporotic Fractures (B-PROOF) trial(155). In the B-PROOF trial, 218 adults aged 65 years and over received a daily oral dose of B vitamins (B12 0·5 mg/d; folic acid 0·4 mg/d) for a period of 2 years. Although the sample did include some adults with a Mini-Mental State Examination (MMSE) score less than 25 (treatment group range, 19–30; placebo group range, 24–30; no significant difference between groups), there were only six such adults in total (3% total population), with four receiving B vitamins. In the absence of other diagnostic tests, it appears that the sample, as a whole, was largely dementia free (summarised in Table 1). Moreover, biochemical analysis indicated that the whole sample had elevated HcY levels (range 12–50 μmol/l).
Analysis of B-PROOF data indicated that HcY and MMA concentrations were reduced, whilst serum concentrations of vitamin B12, folate and holoTC were increased in the adults who received B vitamin supplementation. Contrary to the findings of the VITACOG trial, TCv was significantly lower in the B vitamin group compared with placebo. There was also a non-significant (P=0·07) trend towards lower WM volume in the treatment group. However, it is important to note that MRI scans in the B-PROOF study were only performed post-supplementation, whereas all VITACOG trial analyses(151–153) included MRI at pre- and post-supplementation time points. Subsequently, there is no certainty as to whether there were any differences in cerebral structure between the groups before receiving either B vitamins or placebo. Further, the absence of pre-supplementation scans makes it impossible to determine whether supplementation with B vitamins (B12 and folic acid) modified the trajectory of cerebral decline over the 2-year supplementation period.
Given the apparent association between cerebral structural integrity and cognition, it is possible that B vitamin supplementation, via reducing HcY, benefits cognitive function in older adults. However, in a recent meta-analysis combining eleven large trials and approximately 22 000 participants, Clarke et al. (156) observed that while B vitamin supplementation was efficacious for lowering HcY concentrations, it had no significant effect upon global cognitive function, individual cognitive domains, or cognitive ageing.
Two recent reviews(157,158) have discussed a number of important methodological weaknesses with the meta-analysis of Clarke et al. (156), thereby indicating that Clarke et al.’s(156) conclusion that HcY lowering via B vitamin treatment does not benefit cognitive ageing was premature. For example, Smith & Refsum(158) highlight that cognitive decline could not be measured for most participants in the included trials, as baseline global cognitive measures were unavailable for 76% of 20 431 participants. Moreover, McCaddon & Miller(157) highlight that many of the tests used to assess cognitive function in the included trials (for example, MMSE) were designed to function as screening tests and are not necessarily sensitive enough to detect the small, yet clinically relevant changes in cognitive function over time (or following interventions seeking to slow decline over time). The selection of low-sensitivity tasks probably limited the extent to which differences in cognitive function between treatment groups over time were detectable. The reviews by McCaddon & Miller(157) and Smith & Refsum(158) also discuss the findings from several clinical trials which conflict with Clarke et al.’s(156) interpretation of results. For example, in the Folic Acid and Carotid Intima-media Thickness (FACIT) trial(159), 2 years’ daily supplementation with folic acid (800 µg) appeared to improve memory performance and processing speed in adults aged 50–70 years with elevated HcY (>13 µmol/l). Likewise, de Jager et al. (160) observed that in older adults with MCI demonstrating elevated HcY (>11·3 µmol/l), chronic B vitamin supplementation preserved verbal episodic memory performance, whilst improving semantic memory performance. These conflicting observations indicate that additional, well-designed clinical trials are required to delineate whether B vitamin supplementation is efficacious for modifying cognitive function or slowing the rate of cognitive decline over time in older adults currently without dementia.
It is likely that benefits to cognition following reduced HcY may occur in response to benefits to cerebral structural integrity. Douaud et al. (151) performed Bayesian network analysis on VITACOG trial data, determining a causative chain of events by which B vitamin supplementation reduced the rate of cognitive decline in older adults. Specifically, it was determined that B vitamin supplementation altered plasma vitamin B12 and folate concentrations, with vitamin B12 modifying (lowering) HcY levels. This directly facilitated a reduction in cerebral GM atrophy over time, subsequently slowing the rate of cognitive decline. Future well-designed RCT should investigate these effects further.
Choline and choline-containing glycerophospholipids
Choline is another nutrient whose level of dietary ingestion may exert an influence on cerebral structural integrity in older adults. There are a number of different foods containing choline regularly consumed in most people’s diet. These include red meat (as well as organ meats), poultry, fish, eggs and milk. Vegetables such as tomatoes, potatoes, soya beans and broccoli are also good sources of choline(161,162). Choline in the diet appears to be mostly incorporated within phospholipids, such as the glycerophospholipid (GPL) known as phosphatidylcholine (PC)(161,162). There are also various supplements supplying PC sourced from animal products or vegetables(163).
Supplementation with choline, or GPL containing choline, may benefit cerebral structural integrity via an effect upon HcY concentrations. Choline helps regulate HcY levels through the betaine–homocysteine methyltransferase pathway, whereby choline is initially oxidised to betaine (also obtainable from the diet). Betaine, through a reaction mediated via the enzyme betaine-homocysteine methyltransferase, then donates a methyl group, thereby facilitating the conversion of HcY to methionine(164). Choline and betaine intake have been observed to negatively correlate with HcY concentrations in adults aged 30–55 years(161). Similar observations have been made using data from the Framingham Heart Study. In this later investigation, Cho et al. (162) identified that dietary choline soured from PC was negatively correlated with HcY levels. However, after multivariate adjustment, only choline derived from dietary glycerophosphocholine, phosphocholine and sphingomyelin significantly predicted HcY levels.
Others have sought to reduce HcY through PC supplementation. In males aged 50–71 years with elevated HcY (11·0–23·1 μmol/l), Olthof et al. (164) observed a significant reduction in HcY by 18% after only 2 weeks of daily supplementation with 34·0 g of soyabean lecithin, whereby PC was the only GPL present. As such, it is possible that supplementation with choline or choline-containing GPL (in high enough doses) may benefit cerebral structural integrity in a similar way to B vitamins (i.e. B6, B12 or folic acid)(165).
Although choline intake is negatively related to HcY concentrations, choline has not yet been subject to any RCT investigating its effects upon cerebral structure in older adults. In fact, the only work examining cerebral structure in relation to choline intake is a cross-sectional analysis of Framingham Heart Study data by Poly et al. (166). In this study, Poly et al. (166) observed that in adults aged 36–83 years, greater intake of dietary choline (FFQ) was associated with lower WMH volume.
While human data linking choline intake and cerebral structural integrity are limited, there is some evidence from animal studies suggesting beneficial effects. Crespo et al. (167) administered a daily dose (150 mg/kg) of cytidinediphosphocholine (CDP-choline; the precursor to PC produced de novo via the CDP–choline pathway) to young (aged 12 months) and old (aged 24 months) rodents for 12 months. Crespo et al. (167) identified improved hippocampal morphology in older rodents that received supplementation, with hippocampal cells more closely resembling those of the younger rodents rather than those drawn from the aged control group. Similarly, Qu et al. (168) orally administered low, medium or high doses (0·05 g/kg, 0·1 g/kg or 0·2 g/kg, respectively) of PC derived from Sthenotuethis oualaniensis (purple squid) spawns to adult rodents for a period of 30 d. Before PC administration rodents received an intracerebral injection of amyloid-β25–35, which facilitated impaired hippocampal morphology and cognitive functioning. However, PC supplementation appeared to repair hippocampal cells, while also improving learning and memory, as measured by performance on the Morris water maze.
n-3 Fatty acids
n-3-FA are major components of all cellular membranes, with species such as DHA being especially prevalent within neuronal membranes(169). However, in several studies, the concentration of DHA within GPL of rodent neural tissue has been observed to decrease with age(170,171). Similar observations have been made in human cerebral tissues(172), though other work demonstrates either increases(173), or no change(174) in cerebral DHA concentrations with increasing age. However, the concentrations of DHA within cerebral tissue also appear to be influenced by dietary intake of n-3-FA. While the levels of unsaturation within neuronal tissue is tightly controlled, dietary insufficiency or deficiency of n-3-FA may lead to increased concentrations of n-6 fatty acids (n-6-FA) such as docosapentaenoic acid (n-6-FA variant) in place of DHA(169), though animal data suggest that these effects may be corrected through dietary modification(175). Importantly, while the short-chain n-3-FA α-linolenic acid may be converted to longer-chain n-3-FA such as DHA, the rate of conversion is low, and subsequently, it may be more efficient to consume whole foods or supplements rich in DHA for boosting the concentrations of this n-3-FA in cerebral tissue(176).
Long-chain n-3-FA such as DHA, but also EPA, may be obtained by consuming marine foods such as oily fish, but also through supplementation (for example, fish oil or krill oil). n-3-FA may also be sourced from bovine (cow) or pig brain where they are stored in GPL form(177–179). Moreover, supplementation with n-3-FA derived from marine or terrestrial (i.e. bovine or pig brain) sources has been shown to elevate the concentrations of n-3-FA within both erythrocytes and neuronal tissues(178–183).
Given that fish, especially oily fish, is a rich source of n-3-FA such as DHA, it is plausible that fish consumption in older adults is predictive of cerebral structural integrity. Indeed, higher fish consumption has been correlated with greater GM volume within the orbitofrontal cortex, posterior cingulate, precuneus and hippocampus in cognitively normal older adults(184). Similarly, elevated fish consumption also predicts lower prevalence of subclinical infarcts and greater WM macrostructural integrity in adults aged 60 years and over(185,186).
Other research has examined the extent to which n-3-FA status predicts cerebral integrity in older adults. Greater plasma n-3-FA status has been associated with larger global GM volumes(187) as well as reduced cortical atrophy or GM degradation within medial temporal structures including the hippocampus, parahippocampus and amygdala(188,189). Likewise, a greater concentration of plasma n-3-FA has been correlated with superior WM macrostructural integrity, with studies observing reduced subclinical infarcts and lower WMH volume with elevated n-3-FA concentrations(190,191).
Although plasma concentrations of n-3-FA may be a useful for predicting cerebral structural integrity, one pertinent issue associated with this measure is that it may be influenced by short-term fluctuations in n-3-FA consumption(192). As such, it may not be an accurate reflection of long-term n-3-FA ingestion. Conversely, the concentration of n-3-FA within erythrocyte membranes may be a better reflection of chronic n-3-FA intake(193).
In a study incorporating over 1111 postmenopausal females, aged 65–80 years, Pottala et al. (194) observed an increased TCv of 2·1 cm3 with each 1 sd increase in erythrocyte-bound n-3-FA (DHA + EPA). Similarly, Pottala et al. (194) also observed greater hippocampal volume in older adults with the highest n-3-FA (DHA + EPA) concentrations compared with those with the lowest. In another study, Tan et al. (195) observed lower TCv, as well as increased WMH volume in older adults (mean age 67 (sd 9) years) whose erythrocyte DHA concentrations were in the lowest quartile compared with those whose concentrations were in the higher quartiles (quartile 2 to quartile 4).
Perhaps the most recent study on linking fatty acid status and cerebral structural integrity is Zamroziewicz et al. (196). The authors performed principal components analysis using thirteen different plasma phospholipid-bound fatty acids to delineate whether optimal cerebral structural integrity and cognitive functioning are dependent upon specific fatty acid profiles. Three separate fatty acid profiles were identified. The first profile comprised three n-6-FA and two n-3-FA and was subsequently designated as the long-chain PUFA (LC-PUFA) profile. The second and third fatty acid profiles primarily consisted of n-6-FA, and n-3-FA, respectively. Interestingly, only the LC-PUFA profile was positively associated with microstructural integrity within the fornix. Moreover, LC-PUFA score was positively associated with memory performance, though fornix microstructural integrity appeared to mediate the relationship between LC-PUFA and memory. This appears to support the contention that nutritional intake may influence cerebral structural integrity, and that these effects may further promote cognitive benefits.
Further evidence suggesting that increased n-3-FA intake is neuroprotective comes from a prospective study by Daiello et al. (197). These authors observed greater hippocampal and cortical volumes in cognitively normal adults, as well as in those with MCI and Alzheimer’s disease, who self-reported the use of fish oil supplements over a 3- to 4-year follow-up period (controlling for baseline diagnosis). These results reflect those from a previous RCT whereby cognitively normal adults, aged 50–75 years, were administered a daily n-3-FA supplement for 6 months. Compared with placebo, Witte et al. (198) observed that chronic high-dose n-3-FA supplementation (total n-3-FA dose 2·2 g/d; DHA 880 mg/d; EPA 1320 mg/d) facilitated increased regional cerebral GM volumes as well as improved cerebral microstructural integrity as reflected by increased FA, as well as decreased MD and RD using DTI. More recently, Zhang et al. (199) administered n-3-FA (2000 mg/d algae-derived DHA) to older adults with MCI for 12 months. They identified increased TCv, as well as hippocampal volume, relative to placebo.
The above studies suggest that increasing the intake of n-3-FA may benefit cerebral structural integrity in older adults without dementia. Subsequently, we would anticipate that elevated dietary intake of fatty acids, or foods rich in these lipids, would have an impact upon cognitive function in this cohort. Increased fish consumption (which facilitates elevated blood and tissue n-3-FA concentrations) has been positively correlated with cognitive functioning as well as a reduced risk of dementia with age(200–204). Likewise, greater n-3-FA status appears to predict better executive functioning(190) as well as a lowered risk of cognitive decline(205). Conversely, a poorer intake of n-3-FA (particularly DHA) appears to predict an increased risk of developing dementia and poor cognitive performance(195,203). Similarly, concentrations of n-6-FA have been observed to be higher in older adults demonstrating cognitive decline than those with stable cognitive function over time(205). Likewise, a higher n-6-FA:n-3-FA ratio, reflecting greater n-6-FA consumption relative to n-3-FA, appears to predict an elevated risk of dementia(206).
Polyphenols
Polyphenols are naturally occurring compounds characterised by several hydroxyl groups on aromatic rings(207). They are present in the seeds, root, stem, leaf and fruit portions of many different plants and are commonly consumed within the human diet(208). Based upon their chemical structure, polyphenols are typically organised into two main groups: flavonoids and non-flavonoids(207). The flavonoid class (including flavonols, flavanones, flavones and anthocyanins) are perhaps the most widely studied class of polyphenol regarding potential therapeutic effects upon neurocognitive health and may be sourced from foods such as red grapes, blueberries, cocoa, green tea, citrus fruits and assorted vegetables (for example, onions, leeks, broccoli and celery)(209). Non-flavonoids include the stilbenes, of which resveratrol has been investigated as a potential modifier of neurocognitive health(210–212). Resveratrol is concentrated within the skin of red grapes, groundnuts, blueberries, raspberries and Japanese knotweed(208).
Increased consumption of foods or beverages rich in polyphenols such as chocolate, berries, tea, wine or grape juice appears to benefit cognitive performance in older adults(213–219). Likewise, oral supplementation with resveratrol has been shown to benefit cognitive function in rodents(220), as well as in human subjects aged 50–75 years(210). However, similar to other nutrients discussed in the present paper, there have been relatively few studies examining whether polyphenol supplementation benefits cerebral structural integrity in older adults without dementia.
There are a limited number of RCT that have investigated the effects of chronic resveratrol supplementation on cerebral structural integrity in dementia-free older adults. Witte et al. (210) examined hippocampal macro- and microstructural integrity in a sample of forty-six healthy middle-aged and older adults following chronic resveratrol supplementation (200 mg/d; derived from Japenese knotweed). While Witte et al. (210) identified improved verbal episodic memory performance following resveratrol treatment, no beneficial effects were identified for hippocampal structure. Hunh et al. (211) and Köbe et al. (212) also failed to identify significant benefits to hippocampal structure following resveratrol supplementation (dosage and treatment period were identical to that reported by Witte et al. (210)) in either healthy older adults or those diagnosed with MCI. However, Köbe et al. (212) do report a trend (though non-significant) towards moderate preservation of left anterior hippocampal volume following resveratrol treatment.
There are a number of factors that may explain the absence of significant treatment effects on cerebral structure in the aforementioned RCT. First, in each of these RCT, analysis was restricted to the hippocampus. Beneficial effects may have been apparent if analysis had been expanded to include additional regions known to deteriorate with age. Further, Witte et al. (210) and Huhn et al. (211) recognise the inclusion of healthy older adults and limited intervention period may have prevented identification of benefits to hippocampal structure, as this cohort may not demonstrate markedly reduced cerebral structure in such a short follow-up period. Köbe et al. (212) also failed to identify significant effects upon hippocampal structure despite their inclusion of older adults more susceptible to cerebral deterioration, though they did report a trend towards preservation of hippocampal volume following resveratrol treatment. It is also possible that the small sample sizes may have underpowered the studies for detecting beneficial effects to hippocampal structure (for example, power analysis by Huhn et al. (211) was based upon detecting cognitive effects rather than cerebral effects). Future research investigating resveratrol supplementation as a means of supporting cerebral structure in older adults should consider each of these points.
Despite the available studies on polyphenol supplementation indicating no beneficial effects on cerebral structure, it is still plausible that polyphenol supplementation may be neuroprotective. For example, biochemical and biophysical factors such as elevated concentrations of pro-inflammatory messengers(150,221,222) or reactive oxygen species(223–225), as well as poorer cardiovascular function as indicated by elevated blood pressure/hypertension(226–228) or increased arterial stiffness(229–231), are negatively related to cerebral structural integrity in older adults. However, the consumption of polyphenols such as flavonoids appears to promote anti-inflammatory(232) and antioxidant(233) effects, as well as better cardiovascular outcomes such as improved blood pressures/risk of hypertension(234,235) and reduced arterial stiffness(236). Increased ingestion of resveratrol likewise appears to exert both anti-inflammatory(237,238) and antioxidant effects(238,239), while also facilitating improvements in cardiovascular function such as reduced systolic blood pressure if given in high enough doses(240,241). As such, it is possible that polyphenol supplementation will exert beneficial effects upon cerebral structure if administered in high enough doses and over longer time periods (perhaps 1 year or more) but also in larger samples of older adults who are also at increased risk of cerebral (and cognitive) decline (for example, MCI or AAMI).
Vitamin D
Vitamin D is somewhat different from those nutrients outlined earlier in that it is obtained through direct exposure of skin to sunlight, in addition to dietary intake. However, despite the availability of vitamin D from sunlight exposure, vitamin D deficiency, or insufficiency, is quite common across the world(242,243). Global variations in vitamin D status may be due in part to biological factors such as skin pigmentation, though regional (for example, seasonal variation in light/dark cycles), cultural (for example, adoption of conservative clothing styles) and lifestyle factors (prevalence of indoor employment and leisure or poorer mobility)(242,243) limiting exposure to direct sunlight are also probably involved. For those individuals at risk of limited sunlight exposure, the importance of nutritionally sourced vitamin D becomes paramount. Several different food sources of vitamin D include animal liver, oily fish, egg yolk and shitake mushrooms. Vitamin D is also available from fortified cereals and milk, as well as singular or polynutrient supplements(244).
Regardless of how it is obtained, vitamin D undergoes hydroxylation within the liver, after which it enters circulation as 25-hydroxyvitamin D (25(OH)D)(242). Further modification occurs within the kidneys, whereby 25(OH)D is transformed into 1,25-dihydroxyvitamin D (1,25(OH)2D). Although this later form is the biologically active form of vitamin D which interacts with vitamin D receptors distributed throughout the body, many health effects are better correlated with the 25(OH)D variant(242).
Ensuring optimal vitamin D status may be an important factor for maintaining cerebral health. In older adults without dementia, increased concentrations of amyloid-β as well as proinflammatory messengers have been correlated with poorer cerebral structural integrity, especially WM integrity(150,221,222,245–249). However, increased intake of vitamin D (by diet or injection) appears to facilitate amyloid-β clearance(250–253), as well as elevating anti-inflammatory and lowering pro-inflammatory messengers(250,253–256). Subsequently, we would expect that in vivo concentrations of vitamin D would be correlated with cerebral structure integrity in older adults.
A positive relationship between vitamin D concentrations and cerebral structural integrity has been demonstrated using data from the Gait and Alzheimer Interactions Tracking (GAIT) study. Data from the GAIT study indicate that lower serum 25(OH)D is predictive of increased severity of WM abnormalities(257,258) as well as increased volume of the lateral ventricles, a marker of cerebral atrophy(259) in adults aged 60 years and over. It is important to note that these analyses of GAIT study data do not report excluding adults with dementia; in fact, inclusion criteria allowed MMSE scores to be as low as 10. Similar observations have been made in older adults aged 50–69 years (no cognitive screening is reported) with lower serum 25(OH)D concentrations correlating with greater WMH volume(260).
In addition to WM macrostructure, vitamin D status is also related to cerebral microstructure. In a study of older adults with memory impairment (adults reported subjective memory complaints, though some were identified as having amnestic MCI or probable Alzheimer’s disease), Moon et al. (261) compared the cerebral microstructure of adults with the lowest (n 28) in vivo concentrations of 25(OH)D with those with the highest (n 27) concentrations (mean concentrations 7·89 (sd 1·96) and 22·10 (sd 7·09) ng/ml, respectively). In contrast to adults with the highest concentrations of 25(OH)D, those with the lowest concentrations demonstrated reduced FA in a range of WM regions and tracts. These included the frontal interhemispheric association fibres of the superior and inferior longitudinal fasciculi, the anterior regions of the cingulum bundle, genu of the corpus callosum, as well as projection fibres of the corticospinal tracts, anterior limb of the internal capsule and anterior corona radiata.
It appears that very few studies have investigated the association between vitamin D and cerebral structural integrity in older adults deemed to be without dementia (following cognitive screening). Walhovd et al. (188) examined CT changes across the lifespan in a sample of 281 cognitively normal adults (aged 44–86 years), identifying that higher baseline concentrations of 25(OH)D predicted reduced thinning of right lateral prefrontal cortex over 2 years of follow-up. Likewise, a recent analysis of Framingham Heart Study data determined that vitamin D deficiency, defined as a serum 25(OH)D level below 10 ng/ml, was associated with lower hippocampal volume in middle-aged and older adults(262). Collectively, data from the above studies indicate that elevated vitamin D status may predict greater cerebral integrity in older adults.
With an apparent beneficial relationship between vitamin D and cerebral structural integrity, it follows that vitamin D is correlated with cognitive function. In a recent systematic review(263) low vitamin D status was associated with poorer cognitive performance and an increased frequency of dementia across six prospective studies consisting of adults aged 65 years or older (n 10896). In other analyses, lower vitamin D status was predictive of poorer cognitive performance, increased rate of cognitive decline, as well as an increased risk of developing MCI or dementia(264–266).
Overall, increased skin exposure to direct sunlight or increased consumption of foods rich in vitamin D may boost vitamin D status, thereby benefiting cerebral structural integrity in older adults. These effects may subsequently underpin the cognitive benefits associated with increased vitamin D status. However, the above studies are cross-sectional and may have included those demonstrating dementia symptomology, even if symptoms were only mild. Well-designed, long-duration RCT are required to determine whether increasing the levels of this vitamin through dietary modification (as opposed to that from increased sunlight exposure) is beneficial to cerebral structural integrity in older adults without dementia.
Patterns of nutritional intake
The above studies indicate that increased intake (inferred by FFQ or blood biomarker concentrations) of select nutrients may be beneficial to cerebral structure integrity in older adults. However, examining nutrients in isolation is not an accurate reflection of normal dietary intake, as diets are composed of complex combinations of nutrients. To this extent, several different studies have examined specific patterns or combinations of nutrients and how they relate to cerebral structural integrity in older adults.
In a study involving FFQ and MRI data from fifty-two cognitively normal adults aged 25–72 years, Berti et al. (267) performed principal components analysis in order to determine whether different nutrient patterns (NP) were associated with cerebral structural integrity. Overall, five distinct NP were identified, though only three were associated with cerebral structure across the entire sample. NP-1, comprised of B vitamins (B1, B2, B3, B6 and B9) and several minerals (i.e. Ca, Fe, Mg, P, K and Se), was positively correlated with frontal lobe GM volume. NP-4 (comprised of vitamin B12, vitamin D and Zn) was positively associated with frontal and temporal GM volumes, and negatively correlated with amyloid-β concentrations within the frontal and parietal lobes. Increased consumption of saturated or trans-saturated fats, cholesterol and Na (NP-5) was found to predict poorer GM volumes within frontal and limbic lobes, thereby demonstrating that while nutritional intake may benefit cerebral structure it may also facilitate detrimental effects.
One difficulty with interpreting the results outlined by Berti et al. (267) is that their analysis involved a small sample of participants across a large non-specific age range (i.e. 25–72 years). As such, the results of this study suggest a relationship between nutritional intake and cerebral structure in adults more broadly. However, Bowman et al. (268) examined thirty separate blood biomarkers associated with a range of different nutrients in a sample of 293 adults aged 65 years and older. The authors performed principal components analysis, determining the presence of eight separate nutrient biomarker patterns (NBP). Of these biomarker patterns, NBP-1 (associated with vitamins B1, B2, B6, B12, folate, as well as vitamins C, D and E) predicted greater TCv whereas NBP-8 (associated with trans-saturated fat) was inversely related to TCv. Bowman et al. (268) also observed that NBP-5 (associated with intake of n-3-FA) was inversely associated with WMH volume. Moreover, NBP-1 (vitamins B, C, D and E), NBP-5 (n-3-FA including EPA and DHA), NBP-6 (n-6-FA including arachidonic acid and γ-linolenic acid), NBP-7 (HDL-cholesterol, lutein and uric acid) and finally NBP-8 (trans-saturated fat) were found to correlate with cognitive performance. Specially, better performance in domains such as executive processing, attention and visuospatial functioning were positively associated with NBP-1, -5 and -7, while worse performance in domains such as memory, attentional, language and processing speed were associated with NBP-6 and -8.
In another study, Gu et al. (269) administered a sixty-one-item FFQ to 239 adults (aged 65 years or older) who also underwent diffusion MRI. Gu et al. (269) performed principal component analysis on the FFQ data, identifying six separate NP. Interestingly, only NP-6, composed of n-3 and n-6 PUFA as well as vitamin E, was significantly associated with cerebral microstructural integrity in these adults (specifically the DTI metric FA). Older adults whose NP-6 score was in the highest tertile demonstrated greater FA than those in the middle or lowest tertiles. Importantly, Gu et al. (269) observed that only n-3-FA and vitamin E levels were positively associated with cerebral FA values. In secondary analysis with only 211 dementia-free adults, both NP-6 and n-3-FA remained associated with WM microstructure. Moreover, they demonstrated that cerebral FA scores completely mediated the relationship between NP-6 and cognitive performance.
Adherence to Mediterranean, ‘prudent’ or Western-style diets
In addition to those key nutrients discussed earlier, a number of studies have examined the extent to which adherence to prudent diets such as a MeDI predicts cerebral structural integrity. A MeDI prescribes a high consumption of fruit, vegetables, legumes, whole grains and olive oil, moderate ingestion of fish, low-moderate intake of red wine with meals and low-fat dairy products (primarily as yoghurt and cheese) but also infrequent consumption of animal products (i.e. red meat and milk)(270,271). Likewise, the Mediterranean-DASH (Dietary Approaches to Stop Hypertension) Intervention for Neurodegenerative Delay, or MIND, diet recommends increased intake of fruit, vegetables, grains and fish, moderate intake of low-fat or non-fat dairy products, and low consumption of meat(272). Due to the foods prescribed, greater adherence to either of these diets would ensure heightened ingestion of many of the nutrients already discussed within the present review.
Prudent dietary patterns such as a MeDI and the MIND appear to benefit cognitive functioning when adherence to these diets is increased. A recent systematic review identified that greater adherence to the MeDI appears to facilitate a reduced rate of cognitive decline with age, as well as reduced risk of developing conditions such as MCI or Alzheimer’s disease(273). Greater adherence to a MeDI has also been positively related to working memory and executive performance in middle-aged and older adults(270). The MIND diet has also been related to reduced rate of age-related cognitive decline(274) and risk of developing Alzheimer’s disease(272).
Probably underpinning the apparent cognitive benefits associated with these diets are benefits to cerebral structural integrity. In fact, several studies have observed greater MeDI adherence predicting greater TCv as well as regional GM and WM volumes in addition to elevated CT within frontal, parietal, temporal and occipital zones(275–277). Similarly, Pelletier et al. (278) observed that greater adherence to a MeDI was associated with greater cerebral microstructural integrity after 9 years of follow-up in older adults within the Bordeaux Three-City Study (aged 67–83 years). This was evident as lower MD, RD and AxD scores within WM regions including the corpus callosum, anterior and posterior thalamic radiations, the paracingulate gyrus, cingulum and the parahippocampal fornix. Importantly, Pelletier et al. (278) identified that preserved integrity within the before-mentioned regions was associated with higher cognitive performance.
Though limited, there are also longitudinal data indicating that adherence to a MeDI is beneficial to cerebral structural integrity in older adults. Luciano et al. (279) examined FFQ data derived from approximately 562 healthy older adults of the Lothian Birth Cohort, aged in their early seventies. The extent to which participants adhered to a MeDI was determined at baseline, while MRI was performed 3 and 6 years later, when participants were aged 73 and 76 years, respectively. Luciano et al. (279) observed that a lower MeDI score predicted greater change in TCv over 3 years, even after adjusting for relevant covariates including cognitive impairment during follow-up (indicated by MMSE score).
There are also data correlating specific component scores within a MeDI (or whole foods consistent with MeDI components) with cerebral structural integrity. More frequent fish intake, greater consumption of vegetables, legumes and whole grains, lower consumption of meat/meat products or improved ratio of monounsaturated:saturated fat consumption have been correlated with greater TCv, CT and reduced WMH volumes(184,271,276,277,280). Pelletier et al. (278) observed greater dairy product intake predicting elevated RD and lower FA within the body and genu of the corpus callosum, while moderate alcohol intake (as opposed to abstinence or intensive alcohol consumption) was associated with better microstructural integrity within the same regions associated with dairy product intake. Other studies have observed increased alcohol intake being either beneficial(281–283) or detrimental(284–286) to cerebral structural integrity. However, only Gu et al. (287) appears to have differentiated between alcohol sources (wine, beer or liquor). Importantly, Gu et al. (287) observed that only low to moderate wine consumption was associated with greater TCv compared with no alcohol consumption. Differentiating alcohol sources provides greater accuracy when examining alcohol intake in the context of a MeDI, as this diet prescribes low-to-moderate consumption of red wine (not alcohol in general), due to it being a rich source of polyphenols from red grapes. As such, future work determining whether moderate alcohol consumption, in the context of a MeDI, is beneficial to cerebral structure, should specifically examine wine consumption rather than total alcohol intake.
Another diet, markedly different from a MeDI, is what may be considered a WeDI. A WeDI is typically characterised by high consumption of processed sugars, saturated and trans-saturated fats in addition to Na (salt), but also reduced consumption of fruit and vegetables. In one recent study, Jacka et al. (288) observed that in adults aged 60–64 years, greater adherence to a WeDI predicted smaller hippocampal volume. Conversely, greater adherence to a healthier ‘prudent’ diet (consisting of fresh vegetables, salad, fruit and grilled fish) was associated with larger hippocampal volumes. However, adherence to either diet was not observed to differentially predict hippocampal volume change over 4 years of follow-up. Other work already discussed has indicted that greater intake of trans-saturated fat appears to predict poorer TCv(268) while elevated intake of trans-saturated fat, cholesterol and Na appeared to correlate with reduced GM volumes within frontal and limbic regions in adults aged 25–72 years(267).
More recently, Croll et al. (289) examined the extent to which diet quality was correlated with cerebral structure in a large cohort (n 4213) of dementia-free Dutch adults (mean age 65·7 years). Diet was assessed using an FFQ, with diet quality being determined via level of adherence to official Dutch dietary guidelines (adherence was scored for fourteen items, with overall adherence/diet quality score ranging from 0 to 14). MeDI adherence score was also calculated to allow comparisons with other studies. After adjusting for covariates including age, sex, physical activity, BMI and energy intake, Croll et al. (289) observed that higher dietary quality score (i.e. greater adherence to Dutch dietary guidelines) correlated with larger TCV, greater GM and WM volumes, as well as increased hippocampal volume. Moreover, Croll et al. (289) identified that these effects were associated with intake of a number of food items specified by the guidelines including vegetables, fruit, whole grains, nuts, dairy products, fish, as well as lower intake of sugar-rich beverages. Similar effects were also apparent with increased MeDI adherence. These results build upon those discussed earlier whereby a more ‘prudent’ diet predicts greater cerebral structural integrity in older adults.
On the whole, greater adherence to a ‘prudent’ diet such as a MeDI, as opposed to a WeDI, may facilitate greater cerebral structural integrity in older adults. However, it is important to note that these studies are generally cross-sectional or prospective by design. As such, they cannot infer causality. Ideally, clinical trials whereby participants were prescribed a MeDI (but also comparison diets such as the WeDI or an unchanged diet as a control group) whilst also undergoing pre- and post-intervention structural or diffusion MRI scans would provide greater insight into the potential neuroprotective effects of this diet. However, to date no such trial appears to have been performed.
Limitations and future directions
There is a growing literature indicating that increased intake of select nutrients (inferred by FFQ or blood biomarker concentrations) is associated with cerebral structural integrity in older adults without dementia. However, there are a number of important limitations with the present research that should be considered. One limitation is that the majority of data comes from cross-sectional studies, which cannot infer causality. Moreover, the cross-sectional literature often utilises poorly defined participant samples (for example, ‘dementia free’) using very basic screening measures (for example, MMSE) rather than specific classifications for neurocognitive health (for example, cognitively normal, AAMI or MCI). As indicated earlier, there are differences in cerebral structural integrity between cognitively normal adults and those with conditions such as AAMI or MCI. As such, the association between nutritional intake and cerebral structural integrity may differ between these groups. Likewise, the cross-sectional studies discussed within the present review often report a lengthy delay (sometimes as much as 8 years) between initial nutrient assessment (FFQ or blood draw/biomarker assessment) and MRI. During this time, it is possible that participants changed their diets, thereby confounding the association between nutritional intake and cerebral structure, or developed more severe neurocognitive impairment. Further, when investigating whole diets such as the MeDI, studies have often taken a much more inclusive approach to diet-specific food groups. This is particularly pertinent regarding alcohol intake, with many studies investigating total alcohol consumption while the MeDI specifically focuses on red wine consumption. Moreover, many of these MeDI studies do not examine alternative diets for comparison of effects.
The available RCT typically address the aforementioned issues through use of specific participant samples (for example, MCI) as well as specifying the length of time between MRI measurements. Importantly, RCT also allow causality to be inferred. However, due to cost restraints, RCT often involve small participant samples, potentially underpowering analysis for detecting cerebral effects. Some have also restricted their analysis to highly specific cerebral regions (for example, hippocampus), whilst a more inclusive approach may have allowed benefits to be observed. Future cross-sectional analyses and RCT investigating the association between nutritional intake and cerebral structural integrity should address these methodological issues in order to improve our understanding of the neurocognitive effects associated with nutritional intake.
Summary and conclusion
The human cerebrum undergoes marked deterioration with increasing age, typically between midlife to older adulthood. Declining cerebral structural integrity appears to be a strong predictor of cognitive function in older adults. Further, it appears that conditions such as AAMI or MCI are characterised by more severe cerebral deterioration than that observed during normal ageing.
There is a growing, though currently limited, literature suggesting that increased intake (inferred by FFQ or blood biomarker concentrations) of select nutrients (predominantly vitamins B6, B12, folate, choline, n-3-FA and vitamin D) or adherence to prudent diets (for example, MeDI) supports cerebral structure in older adults without dementia. There is also a growing number of RCT indicating that nutritional supplementation, particularly B vitamins (for example, B6, B12 and folic acid) or n-3-FA may be neuroprotective. However, these studies do contain a number of important limitations discussed earlier, which should be addressed in future work.
Overall, the available literature suggests that elevated ingestion of select key nutrients, or greater adherence to specific diets (for example, the MeDI), may benefit cerebral structural integrity in older adults. Some studies have even indicated that cerebral structural integrity may mediate the relationship between nutritional intake and cognitive function. As such, nutritional modification may be a readily implementable therapeutic intervention, capable of supporting neurocognitive health with age. If these effects can be confirmed in larger, well-designed RCT, community attitudes regarding long-term dietary behaviours may begin to change. Not only would this contribute to maximising our own neurocognitive health as we age, but society as a whole may benefit from lower incidence of age-related neurodegenerative diseases such as dementia.
Acknowledgements
J. M. R. drafted the manuscript. All authors were actively involved in the planning and the development of the manuscript. J. M. R. receives a Research Training Program Stipend as part of his postgraduate studies. J. M. R. declares no potential conflicts of interest.
H. M. has received research funding from the National Health and Medical Research Council, Australian Research Council, Alzheimer Australia grants as well as from Swisse Wellness.
D. J. W. has received research funding and/or consultancy/speaker fees from Abbott Nutrition, Arla Foods, Bayer Healthcare and Neurobrands.
A. S. has received research funding and/or consultancy/speaker fees from a number of food industry partners including Abbott Nutrition, Arla Foods, Australian Wine Research Institute, Barilla, Bayer Healthcare, Blackmores, Cognis, Cyvex, Dairy Health Innovation Consortium, Danone, Ginsana, GlaxoSmithKline Healthcare, Masterfoods, Martek, Naturex, Nestlé, Novartis, Red Bull, Sanofi, Unilever, Verdure Sciences and Wrigley.
A. P. has received research funding and/or consultancy/speaker fees from Biostime, Blackmores, DSM, LifeVantage, Novasel Australia, Enzo Nutraceuticals and Swisse Wellness. A. P. was previously a member of the Scientific Advisory Panel for Swisse Wellness.
The present study was not supported by any specific grant from any funding agency, commercial or not-for-profit entity.
Supplementary material
The supplementary material for this article can be found at https://doi.org/10.1017/S0954422418000185