Introduction
Ageing is a natural biological process that impacts every living organism, affecting various aspects of physical, mental and emotional health owing to intricate physiological and dynamic changes over time. Most gerontologists assert that it starts in the fourth decade of life and leads to death. This complex and individual process takes place on a biological, psychological and social level. The biological process of ageing causes progressive changes in metabolism and physico-chemical properties of cells, resulting in impaired self-regulation, regeneration and structural and functional changes. This is a natural, irreversible process that can be successful, typical or pathological. The biological changes due to ageing affect moods, attitudes towards the environment, physical conditions and social activity, as well as seniors’ position in their families and communities. A person’s psychological ageing is determined by their awareness and their ability to adapt to the ageing process. The following adaptation attitudes can be distinguished: constructive, dependent, hostile and self-centred. A person’s ability to adjust to a new situation deteriorates with age, cognitive and intellectual changes occur, perception processes deteriorate, perceived sensations and information are lowered and thinking processes change. Social conditioning constrains the function of the elderly to that of senior citizens, and traditions may evolve over time. Social ageing refers to the way a human being perceives and experiences the ageing process(Reference Dziechciaż and Filip1).
Researchers have long been fascinated by ageing, leading to a search for interventions that not only extend lifespan but also maintain health and vitality in old age. To explore potential interventions for healthy ageing, it is essential to understand the ageing process itself, which is governed by genetic and cellular mechanisms(Reference Farr and Almeida2). At the genetic level, ageing is driven by accumulated DNA damage,(Reference Sourada and Kuglík3) resulting from environmental pollutants, radiation and errors in DNA replication over time. These mutations impair cellular function and contribute to ageing(Reference Khrapko and Turnbull4). Telomere shortening is another key factor in ageing(Reference Fathi, Charoudeh, Sanaat and Farahzadi5). Telomeres protect chromosome ends during cell division, but as they shorten, cells lose their ability to divide effectively, leading to cellular senescence and a decline in tissue regeneration(Reference Turner, Vasu and Griffin6). Oxidative stress, caused by the accumulation of reactive oxygen species (ROS), plays a major role in ageing(Reference Jiang, Xu, Li, Xia, Wang and Yang7,Reference Coluzzi, Leone and Sgura8) . by damaging proteins, DNA and lipids, leading to ageing-related changes in tissues and organs(Reference Lundgren, Sjöstrand, Biner, Bennett, Rudling and Johansson9). Various tissues and organs show ageing-related changes as a result of oxidative stress(Reference Labunskyy and Gladyshev10). As mitochondria, the cell’s energy producers, become less efficient with age, they generate more ROS, further contributing to cellular damage(Reference Giorgi, Marchi, Simoes, Ren, Morciano and Perrone11). Ageing is also influenced by chronic inflammation(Reference Franceschi and Campisi12). Chronic inflammation, resulting from persistent immune system activation, is closely linked with age-related diseases(Reference Akazawa13). Age-related changes in gene expression further accelerate ageing, with genes responsible for repair and maintenance becoming less active, while those associated with inflammation and stress responses become more active(Reference Harris, Riggio, Evenden, Gilchrist, McCafferty and Murphy14). By changing gene expression, ageing can be accelerated. Another hallmark of ageing is protein misfolding and aggregation(Reference Cuanalo-Contreras, Mukherjee and Soto15). Protein misfolding and aggregation, which interfere with cellular function, are also hallmarks of ageing and are implicated in neurodegenerative diseases such as Alzheimer’s and Parkinson’s. In Alzheimer’s disease, β-amyloid and tau proteins are misfolded in the brain. Typically, Parkinson’s disease is characterised by brain accumulations of α-synuclein protein(Reference Forloni, Terreni, Bertani, Fogliarino, Invernizzi and Assini16).
Hormonal changes, such as declining levels of insulin-like growth factor (IGF-1), growth hormone (GH) and sex hormones, also contribute to the ageing process(Reference Copinschi and Caufriez17). Table 1 summarises ageing factors.
Table 1. Factors associated with ageing
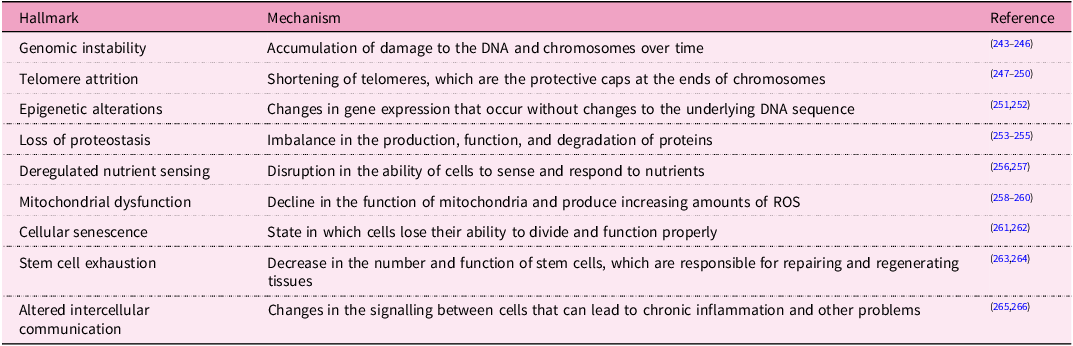
Research into lifespan and healthy ageing has increasingly focused on natural preventative factors. Compounds including omega-3 fatty acids, coenzyme Q10, gingerol (from ginger) and curcumin (from turmeric) have emerged as potential anti-ageing agents, (Fig. 1) offering benefits such as reduced inflammation, improved cardiovascular health and enhanced cognitive function. The selection of these supplements was based on their well-documented effects on the ageing process, particularly in mitigating oxidative stress, inflammation and mitochondrial dysfunction, which are common contributors to ageing. Although each compound acts through different molecular mechanisms, they share a unifying link in promoting cellular health and longevity. For instance, omega-3s and coenzyme Q10 support cardiovascular and cognitive health, whereas gingerol and curcumin are powerful anti-inflammatory and antioxidant agents. These compounds may also lower the risk of cancer and dementia associated with ageing. By examining current scientific studies, we can explore how these natural substances contribute to healthy ageing and longevity through their complex molecular mechanisms, providing valuable insights into their potential as preventative factors. Research has been undertaken on the compounds present in certain foods through scientific studies. In foods, bioactive compounds are extra-nutritional components found in small quantities. A number of bioactive compounds appear to be beneficial to health. For example, there is evidence that consuming foods rich in flavanones (naringenin) and flavanols (quercetin) reduces cardiovascular damage and tumour growth(Reference Sánchez Macarro, Martínez Rodríguez, Bernal Morell, Pérez-Piñero, Victoria-Montesinos and García-Muñoz18–Reference Asgharian, Tazekand, Hosseini, Forouhandeh, Ghasemnejad and Ranjbar20). Oxidative stress contributes to various disorders, including cancers, metabolic disorders, inflammatory condition, cardiovascular diseases and brain disorders(Reference Abdolmohammadi, Mahmoudi, Alimohammadi, Tahmasebi, Zavvar and Hashemi21–Reference Zare Rafie, Esmaeilzadeh, Ghoreishi, Tahmasebi, Faghihzadeh and Elahi24). There are several epidemiological studies suggesting that polyphenol compounds in foods, such as flavonoids, phenolic acids, lignans, stilbenes, tannins and anthocyanins, may delay the onset of degenerative diseases(Reference Liu25,Reference Tressera-Rimbau, Arranz, Eder and Vallverdú-Queralt26) . We will look at the current research to explore how these natural substances may serve as preventative factors. This review explores the role of omega-3, coenzyme Q10, gingerol and curcumin in ageing through a scientific exploration of the scientific literature. Furthermore, we will investigate how these substances exert their effects through complex molecular mechanisms. As a result, we hope to provide comprehensive insights into the potential for healthy ageing and longevity using these natural compounds.
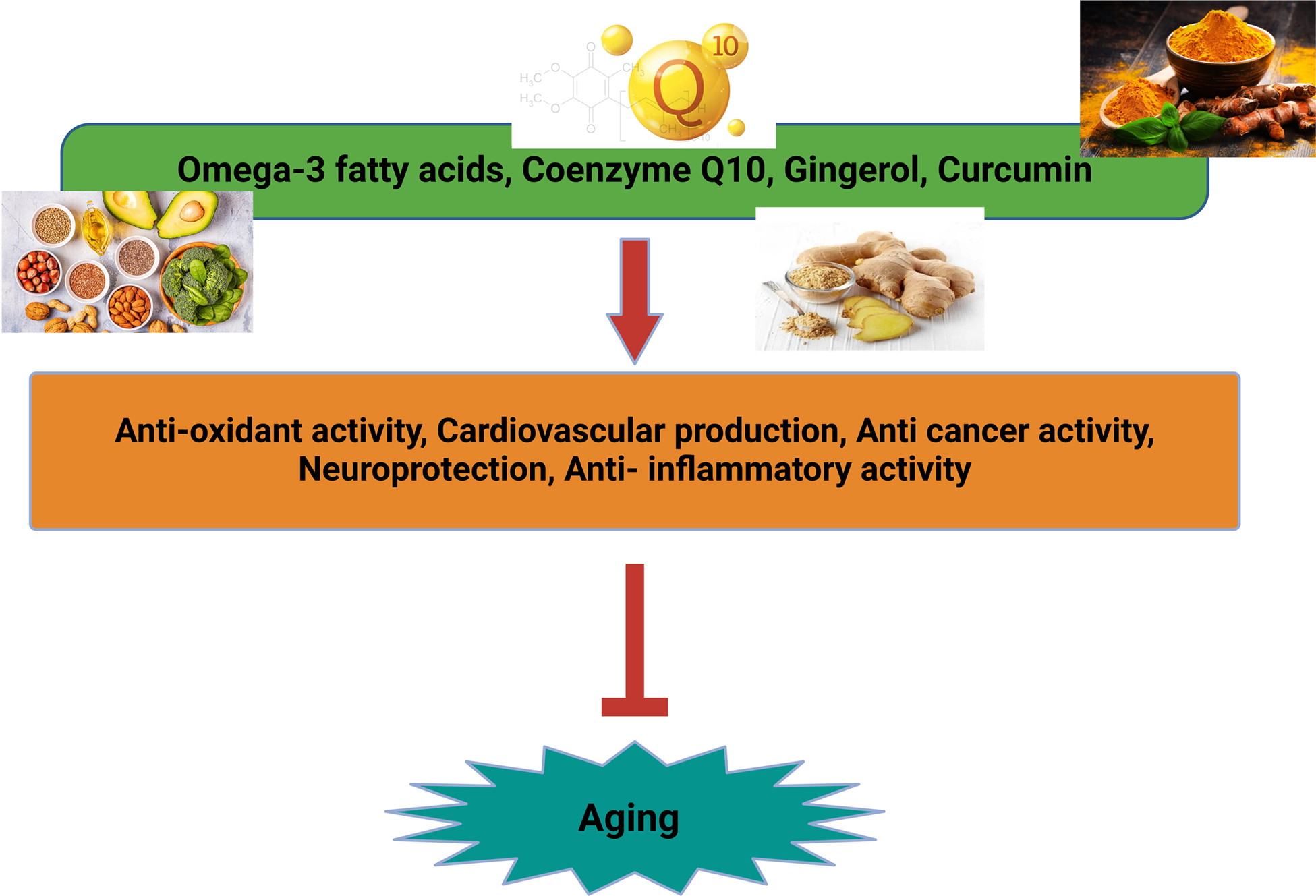
Figure 1. A schematic showing the anti-ageing effects of omega-3, coenzyme Q10, gingerol and curcumin.
Omega-3
Many health benefits can be attributed to omega-3 fatty acids, which are polyunsaturated fatty acids (PUFA). They can help reduce inflammation(Reference Calder and Grimble27) as well as the risk of heart disease, stroke(Reference Ander, Dupasquier, Prociuk and Pierce28,Reference Endo and Arita29) , certain types of cancer(Reference Paixão, Oliveira, Pizato, Muniz-Junqueira, Magalhães and Nakano30,Reference Chagas, Borges, de Oliveira, Mocellin, Barbosa and Camargo31) , rheumatoid arthritis(Reference Simopoulos32), depression(Reference Su, Tseng, Lin, Okubo, Chen and Chen33) and asthma(Reference Brigham, Woo, McCormack, Rice, Koehler and Vulcain34). Omega-3 fatty acids are also essential for brain health(Reference Dighriri, Alsubaie, Hakami, Hamithi, Alshekh and Khobrani35), helping to maintain cognitive function(Reference Welty36) and reduce the risk of dementia(Reference Wei, Li, Dong, Tan and Xu37). They play an important role in human physiology, but the body cannot synthesise them, so they must be obtained from food or supplements. Table 2 summarises the adequate amount of omega-3. Omega-3 fatty acids come in three forms: eicosapentaenoic acid (EPA), α-linolenic acid (ALA) and docosahexaenoic acid (DHA)(Reference Krupa, Fritz and Parmar38). Plant sources of ALA include rapeseed, soybean, flaxseed and walnut oils, as well as dark-green leafy vegetables, soy, chia seeds and walnuts. Fish and fish oil contain DHA and EPA, especially cold-water fish such as tuna, salmon, trout, mackerel, whitefish and herring(Reference Jacobsen39). In addition to triacylglycerols, omega-3 fatty acids are available as phospholipids, acylglycerols and ethyl esters(Reference Shahidi and Ambigaipalan40). Gastric lipases digest omega-3 fatty acids and convert them into diacylglycerol and fatty acids in the stomach(Reference Shahidi and Ambigaipalan40,Reference Ito41) . In the small intestine, pancreatic lipases and bile salts further break them down. Pancreatic carboxylic acid ester lipase breaks down omega-3 ethyl esters into EPA and DHA(Reference Shahidi and Ambigaipalan40). Micelles are absorbed by enterocytes and transported by fatty acid transport proteins. Following re-esterification, they are converted into triacylglycerols and enter the circulatory system as chylomicrons(Reference Schuchardt and Hahn42,Reference Innis43) . β-Oxidation is the primary pathway for the metabolism of DHA and EPA, with cytochrome P450 (CYP) playing a minor role(Reference Yang, Yi, Zhang, Li, Lang and Ling44). Omega-3 fatty acids are bioavailable based on several factors, including their form and meal content. As a general guideline, bioavailability is ranked from highest to lowest based on lipid structure (re-esterified triacylglycerols, NEFA, ethyl esters, phospholipids, triacylglycerols and so on)(Reference Cholewski, Tomczykowa and Tomczyk45). Phospholipids have higher bioavailability than other forms, and krill oil has high bioavailability compared with other marine sources(Reference Schuchardt and Hahn42). There is debate on how the chemical positioning of omega-3 fatty acids in oils affects bioavailability, with some studies suggesting that different positions may be more effective(Reference Cholewski, Tomczykowa and Tomczyk45). The average American diet is significantly below the recommended levels of EPA and DHA. EPA and DHA can be obtained from dietary supplements, but many of these products contain ethyl ester formulations that are not well absorbed without a meal containing fat. The in situ emulsification of EPA and DHA enhances their absorption, thereby improving their bioavailability regardless of the presence of fats in the meal. Omega-3 fatty acid supplements containing absorption enhancers have been shown to significantly increase the bioavailability of EPA and DHA in randomised controlled trials(Reference Maki and Dicklin46).
Table 2. The Adequate Intakes of Omega-3(Reference Trumbo, Schlicker, Yates and Poos267)
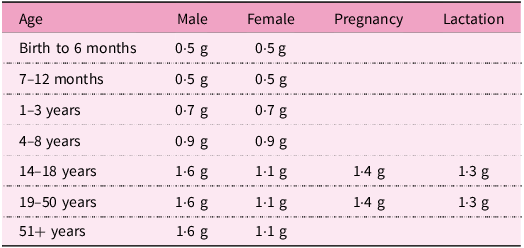
To maintain good health, adults should consume 250–500 mg of combined EPA and DHA per day. There is no official recommended daily allowance for DHA and EPA(Reference Vannice and Rasmussen47,48) . Some health conditions, however, require higher amounts. The American Heart Association recommends omega-3 supplements containing EPA and DHA daily to people with coronary heart disease or heart failure and recommends 4000 mg/d of omega-3 supplements for people with high triacylglycerol(49,Reference McDonnell, French, Baggerly and Harris50) .
Omega-3 supplements are generally considered safe. The Food and Drug Administration (FDA) recommends consuming no more than 5 g of EPA and DHA combined per day from dietary supplements(Reference Zhang, Fulgoni, Kris-Etherton and Mitmesser51). Some omega-3 supplements may cause minor side effects, such as odorous breath, nausea, fishy aftertaste, diarrhoea and stomach cramps(Reference Mazereeuw, Lanctôt, Chau, Swardfager and Herrmann52,Reference Lev-Tzion, Griffiths, Leder and Turner53) . Omega-3 deficiencies can lead to rough, scaly skin and dermatitis(Reference Bjerve, Fischer, Wammer and Egeland54). A variety of inflammatory pathways have been shown to be influenced by omega-3 fatty acids(Reference Sakamoto, Saotome, Iguchi and Maekawa55–Reference Krupa, Fritz and Parmar58), including restricting the movement of leucocytes towards inflammation sites (leucocyte chemotaxis inhibition)(Reference Calder59); reducing the expression of adhesion molecules, particularly those involved in interactions between leucocytes and blood vessel walls (leucocyte–endothelial adhesive interactions)(Reference Sethi, Ziouzenkova, Ni, Wagner, Plutzky and Mayadas60); suppressing the activity of cyclooxygenase (COX) and the subsequent production of eicosanoids such as leukotrienes and prostaglandins derived from arachidonic acid(Reference Calder59,Reference Calder61) ; reducing the levels of proinflammatory cytokines such as IL-1β, TNF-α and IL-6(Reference Nielsen, Jørgensen, Nielsen, Eivindson, Grønbaek and Vind62); increasing the production of compounds that resolve inflammation, such as maresins, protectins, resolvins and lipoxins(Reference Serhan and Levy63); inhibiting NF-kB, a transcription factor that promotes inflammation(Reference Calder57); activating the anti-inflammatory transcription factor NR1C3 (Reference Calder57); activating peroxisome proliferator-activated receptors (PPAR) that have anti-inflammatory effects(Reference Calder59); inducing anti-inflammatory effects by activating GPR120, a G-protein-coupled receptor(Reference Wellhauser and Belsham64); and disrupting lipid rafts in cell membranes and altering phospholipid composition(Reference Hashimoto, Hossain and Viduranga65–Reference de Carvalho and Caramujo67). Normal brain function and ageing depend on omega-3 fatty acids. They can reduce inflammation and protect against cognitive decline and dementia. Omega-3 has been studied extensively in relation to ageing and longevity. Several studies have shown that it can slow down the ageing process and extend life. Several studies suggest that omega-3 may protect against dementia and cognitive decline associated with ageing. Observational studies and clinical trials have provided conflicting results regarding the efficacy of omega-3 fatty acid supplementation for preventing cognitive decline, dementia or Alzheimer’s disease (AD). According to prospective studies, individuals with higher omega-3 fatty acid intakes are less likely to develop AD(Reference Wood, Chappell and Zulyniak68), and erythrocyte DHA levels may be inversely associated with the risk of AD and dementia of any cause(Reference Sala-Vila, Satizabal, Tintle, Melo van Lent, Vasan and Beiser69). In comparison with cognitively healthy individuals, patients with AD have lower serum omega-3 fatty acid concentrations, plasma phospholipids and erythrocyte membrane omega-3 fatty acid concentrations(Reference Tully, Roche, Doyle, Fallon, Bruce and Lawlor70,Reference Whalley, Deary, Starr, Wahle, Rance and Bourne71) . The effects of omega-3 fatty acid supplementation on cognitive decline and probable AD, however, have been limited in randomised clinical trials(Reference Phillips, Childs, Calder and Rogers72). It is also possible that the genotype of apolipoprotein 4 (APOE ε4) might influence the relationship between omega-3 fatty acid supplementation and dementia, or cognitive decline and AD(Reference van de Rest, Wang, Barnes, Tangney, Bennett and Morris73,Reference Li, Xu, Tan, Cao, Wei and Dong74) . The APOE ε4 allele, the major genetic risk factor for AD, could induce abnormal cholesterol metabolism as part of the AD-associated pathology(Reference Jeong, Lee, Cho and Seo75). There is still a lack of information about how APOE ε4 interacts with omega-3 fatty acids to affect dementia risk and cognitive decline. A study found an association between omega-3 fatty acid consumption and APOE ε4 carriers, but not APOE ε4 non-carriers, experiencing slower cognitive decline(Reference van de Rest, Wang, Barnes, Tangney, Bennett and Morris73). According to other studies, omega-3 fatty acids are beneficial only for APOE ε4 non-carriers(Reference Whalley, Deary, Starr, Wahle, Rance and Bourne71,Reference Beydoun, Kaufman, Satia, Rosamond and Folsom76) . A possible explanation for this may be that APOE is reducing DHA and EPA delivery to the brain(Reference Barberger-Gateau, Samieri, Féart and Plourde77). However, findings from a meta-analysis suggest that (1) long-term omega-3 fatty acid supplementation may reduce the risk of AD; (2) dietary intake of omega-3 fatty acids, particularly DHA, may reduce dementia and cognitive decline risks; and (3) peripheral biomarkers of omega-3 fatty acids may be able to predict cognitive decline(Reference Wei, Li, Dong, Tan and Xu37). Nevertheless, additional research is needed to explore the gene–environment interactions associated with omega-3 fatty acid intake. According to research by Wenbo Qi et al., consuming polyunsaturated fatty acids reduces the risk of ageing-related diseases, especially cardiovascular disease and stroke. Researchers found alterations in lipid metabolism, specifically an increase in ALA synthesis, in long-lived Caenorhabditis elegans glp-1 germline-less mutants. In C. elegans, ALA extends lifespan in a dose-dependent manner. In addition, the extended longevity observed in the glp-1 mutant animals relies on two crucial transcription factors, NHR-49/PPARα and SKN-1/Nrf2, although the exact mechanisms are not fully understood. Both NHR-49 and SKN-1 transcription factors are required for ALA treatment to prolong the lifespan of wild-type worms. ALA activates NHR-49 and upregulates genes involved in lipid β-oxidation. ALA does not directly activate SKN-1; however, exposure to air results in the formation of oxylipins. In addition to extending lifespan, ALA treatment activates one of these oxylipins. Several studies have documented the anti-ageing effects of omega-3 fatty acids and their oxylipin metabolites. In addition, oxylipins may contribute to the health benefits associated with consuming omega-3 fatty acids. There are differences between observational and interventional clinical trials regarding the effects of omega-3 fatty acid intake on human health due to variations in oxylipin conversion(Reference Qi, Gutierrez, Gao, Dixon, McDonough and Marini78).
Another study in 2023 found that a structured triacylglycerol form of DHA (DHA-TG) had a positive impact on the health span of aged C. elegans, focusing on cognitive health. DHA-TG improved the nematodes’ mobility at various stages of adulthood without affecting their overall lifespan. The treatment also activated superoxide dismutase in the nematodes, increasing their antioxidant capability. This study suggests that the DAF-16/FOXO transcription factor might be involved in DHA-TG’s beneficial effects, suggesting an intermediary in DHA’s mechanism of action. DHA-TG bolstered antioxidant defences and significantly increased physical fitness in ageing C. elegans, which could have implications for cognitive function(Reference Mora, Pérez-Santamaria, Tortajada-Pérez, Vázquez-Manrique, Arola and Puiggròs79).
A study conducted by Kamil M. and his colleagues on Drosophila demonstrated that dietary supplements containing monoacylglycerol n-3 PUFA can prolong the lifespan of male Drosophila flies. These supplements not only affect mitochondrial oxidative capacity but also influence thoracic muscle markers related to oxidative stress(Reference Champigny, Cormier, Simard, St-Coeur, Fortin and Pichaud80).
The omega-3 fatty acid EPA was studied in 2017 in response to saturated fat and inflammation in mouse skeletal muscle cells. The study found that adding EPA under cytotoxic stress partially rescued differentiation, with increased expression of MyoD, myogenin, IGF-II and IGFBP-5 associated with enhanced myotube formation. In simpler terms, the study showed that EPA can improve the ability of muscle cells to regenerate even when exposed to harmful conditions such as inflammation and saturated fat(Reference Saini, Sharples, Al-Shanti and Stewart81).
The hippocampus, a region crucial to memory and learning, has been shown to reverse age-related changes when supplemented with n-3 fatty acids, specifically DHA. Levels of GluR2 and NR2B, two glutamate receptor subunits, decrease in ageing rats. However, when aged rats are given n-3 fatty acid supplementation, these levels return to those seen in young adult rats(Reference Su82). Fish oil supplementation has been shown to extend the lifespan of female mice prone to autoimmune disorders by more than 40%(Reference Jolly, Muthukumar, Avula, Troyer and Fernandes83,Reference Fernandes84) . Several cardiac dysfunctions have been attenuated by fish oil rich in omega-3 fatty acids in ageing mice, including hypertrophy of the ventricular wall and remodelling of the cardiac muscle(Reference Halade, Williams, Lindsey and Fernandes85). It has been shown that omega-3 PUFA EPA and DHA can reverse the decline in retinoid receptors and PPARγ in aged animals and may improve neurogenesis(Reference Dyall, Michael and Michael-Titus86). The research conducted by Qureshi and his team found that middle-aged and older rats were inhibited or prevented from releasing senescent microvesicles (SMV) when administered EPA at a ratio of 6:1, which are associated with pro-senescent, pro-thrombotic and pro-inflammatory effects on endothelial cells. The local angiotensin system likely facilitates this influence. Endothelial dysfunction may be delayed by maintaining an EPA ratio of 6:1(Reference Qureshi, Altamimy, El Habhab, El Itawi, Farooq and Zobairi87). Specific and relevant changes in glutamatergic transmission underlie the potent neuroprotective effects of omega-3 PUFAs during ageing in the central nervous system. Through improved glutamatergic transmission, omega-3 can protect the central nervous system during ageing(Reference Dyall, Michael, Whelpton, Scott and Michael-Titus88). Telomere length is one of the important hallmarks of ageing(Reference Razgonova, Zakharenko, Golokhvast, Thanasoula, Sarandi and Nikolouzakis89,Reference Epel, Blackburn, Lin, Dhabhar, Adler and Morrow90) . Various doses of omega-3 fatty acids were administered to male mice over 2 months in one study, and it was found that both high-dose and low-dose DHA reduced hepatic telomere shortening, while fish oil and low-dose DHA inhibited the attrition of testicular telomeres(Reference Chen, Wei, Chen, Jiao and Zhang91). Research suggests that PUFA may promote telomere length by counteracting age-related increases in TRF-1 expression, as observed in pigs supplemented with linseed oil for 9 weeks(Reference Ogłuszka, Te Pas, Poławska, Nawrocka, Stepanow and Pierzchała92). Having omega-3 fatty acids in the diet reduced the rate of telomere attrition and elongated the telomeres of rats in another study(Reference Varela-Lopez, Pérez-López, Ramirez-Tortosa, Battino, Granados-Principal and del Carmen Ramirez-Tortosa93). Comparative studies on telomere length showed that fish oil containing 60% omega-3 PUFA had a positive effect compared with control fish oil(Reference Gao, Xiao, Li, Guo, Cai and Li94). Another study examined the role of fish oil, DHA and arachidonic acid in mice whose ageing was induced by d-galactose. In this study, fish oil, DHA and arachidonic acid were linked to ageing via a redox–telomere–antioncogene axis. In addition to improving redox balance, reducing oxidative stress and protecting against telomere shortening, omega-3 and fish oil appear to be beneficial. Interestingly, n-3 PUFA, particularly DHA, suppress cellular senescence pathways, demonstrating their anti-ageing potential(Reference Chen, Wei, Chen, Jiao and Zhang95).
In conclusion, the research presented here highlights the significant positive effects of ω-3 polyunsaturated fatty acids, particularly ALA and DHA, on various aspects of health and ageing. These studies demonstrate that ALA can extend lifespan, potentially through NHR-49/PPARα and SKN-1/Nrf2 transcription factors, and that DHA, especially in structured triacylglycerol form (DHA-TG), can improve health span by enhancing mobility and antioxidant defences(Reference Qi, Gutierrez, Gao, Dixon, McDonough and Marini78). It has been demonstrated that omega-3 fatty acids, such as EPA, support muscle cell regeneration even when harmful factors are present. In addition, omega-3 PUFA supplementation promotes neuroprotection by reversing age-related changes in the hippocampus and improving glutamatergic transmission. According to these findings, omega-3 fatty acids may play an important role in promoting health and longevity through a variety of molecular mechanisms.
Extensive research has explored the role of omega-3 fatty acids in ageing and age-related diseases in humans. A significant amount of research has been conducted in the field of ageing and longevity on telomeres, the protective caps at the ends of chromosomes. Often considered markers of biological ageing, these structures are crucial for cellular division. One area of interest to researchers has been the possible impact of omega-3 fatty acids on telomere length. Many studies have investigated the relationship between omega-3 fatty acid intake and telomere length to discover whether these essential fatty acids maintain telomere health(Reference Ogłuszka, Lipiński and Starzyński96). The telomere length of patients with coronary heart disease may be protected by marine omega-3 fatty acids(Reference Farzaneh-Far, Lin, Epel, Harris, Blackburn and Whooley97). According to Liu et al. (2021), preschool children with obesity who consumed more omega-3 fatty acids had longer telomeres and lower levels of telomerase methylation(Reference Liu, Shi, Fan, Chen, Chen and Zhao98). Omega-3 fatty acids may protect telomere length from oxidative stress, according to a randomised controlled trial(Reference Kiecolt-Glaser, Epel, Belury, Andridge, Lin and Glaser99). Researchers have found that omega-3 fatty acids may play a protective role in maintaining the length of the telomeres of leukocytes in patients with chronic kidney disease(Reference Barden, O’Callaghan, Burke, Mas, Beilin and Fenech100). Among older individuals with mild cognitive impairment, omega-3 fatty acid supplementation reduced the shortening of telomeres by 23% in a preliminary study conducted by O’Callaghan et al. in 2014(Reference O’Callaghan, Parletta, Milte, Benassi-Evans, Fenech and Howe101). Ongoing research continues to explore the exact mechanisms and implications of omega-3 fatty acids and telomere length, although these studies collectively suggest a link.
Sedentary, overweight, middle-aged participants in a 2021 study were assessed for cellular ageing-related biomarkers following omega-3 supplementation. Using the Trier Social Stress Test, participants were randomly assigned to receive 2·5 g of omega-3 every day, 1·25 g of omega-3 every day or a placebo for a 4-month period. Telomerase and interleukin-10 stress reactivity were influenced by omega-3 supplementation. In both supplementation groups, telomerase and interleukin-10 levels were protected from declines following stress. Omega-3 at 2·5 g/d reduced overall cortisol and interleukin-6 levels, showing a significant decrease compared with the placebo group. By reducing inflammation and cortisol levels during stress and enhancing repair mechanisms during recovery, omega-3 may be able to slow down the ageing process and lower depression risk(Reference Madison, Belury, Andridge, Renna, Rosie Shrout and Malarkey102). EPA, DPA and DHA were found to increase the chances of older individuals experiencing a robust and healthy ageing process, according to a prospective cohort study conducted by Heidi TM Lai and colleagues(Reference Lai, de Oliveira Otto, Lemaitre, McKnight, Song and King103). Harris et al. found that higher circulating levels of marine omega-3, including eicosapentaenoic, docosapentaenoic and docosahexaenoic acids, were associated with a significant reduction in premature mortality, as confirmed by an analysis of data from seventeen prospective cohort studies(Reference Harris, Tintle, Imamura, Qian, Korat and Marklund104).
Studies conducted in 2013 found lower total mortality, particularly cardiovascular mortality, in people with higher plasma levels of omega-3 polyunsaturated fatty acid biomarkers(Reference Mozaffarian, Lemaitre, King, Song, Huang and Sacks105). Over a 3-year period, PUFA levels in the blood, likely reflecting dietary intake, were associated with better physical performance maintenance and a reduced risk of decline in the InCHIANTI study in older Italians. Alternatively, higher levels of n-6 PUFA were associated with poor physical performance and slowed walking speed. Physical performance can be preserved as individuals age through dietary fatty acids, especially n-3 PUFA(Reference Abbatecola, Cherubini, Guralnik, Andres Lacueva, Ruggiero and Maggio106).
There is growing evidence that PUFA and their compounds can help combat cognitive decline related to ageing. Fish oil supplements totalling 0·4 g of EPA and 2 g of DHA significantly improved cognitive processing speed in middle-aged to older adults with obesity(Reference Kuszewski, Howe and Wong107). EPA and DHA daily doses of 1·86 g and 1·5 g were beneficial to patients with coronary artery disease and ischaemic risk over 30 months, leading to improved cognitive function(Reference Malik, Ramadan, Vemuri, Siddiq, Amangurbanova and Ali108). A second study found that taking 1·6 g of EPA and 0·8 g of DHA daily for 24 weeks reduced cognitive inefficiency in daily activities in older adults with no risk factors but self-perceived cognitive impairment. In a double-blind, randomised controlled trial, fish oil was shown to enhance memory function in individuals with mild cognitive impairment (MCI)(Reference Gillies, Leach and Algorta109). According to a 2010 study, supplementing with 900 mg of DHA for 24 weeks improved learning and memory in older adults with age-related cognitive decline (ARCD)(Reference Yurko-Mauro, McCarthy, Rom, Nelson, Ryan and Blackwell110). According to a meta-analysis by Bao-Zhen et al., omega-3 polyunsaturated fatty acid intake is associated with cognitive impairment, AD and dementia(Reference Wei, Li, Dong, Tan and Xu37).
Overall, fish oil supplements with varying EPA and DHA content appear to enhance cognitive function in individuals with diverse conditions, including coronary artery disease, obesity and age-related cognitive impairments. Age-related macular degeneration (AMD) mainly affects older adults and can cause severe vision loss. The macula is the central component of the retina, responsible for sharp, central vision, which is affected by AMD(Reference Iroku-Malize and Kirsch111). Due to their potential health benefits for the eyes, omega-3 fatty acids have been a topic of interest in relation to AMD. Researchers found that DHA and EPA consumption significantly reduced the risk of AMD development in 235 patients with AMD, following them for 10 years(Reference Christen, Schaumberg, Glynn and Buring112). Augood and Seddon’s study supports the recent findings(Reference Augood, Chakravarthy, Young, Vioque, de Jong and Bentham113,Reference Seddon, George and Rosner114) . A review of human and animal research suggests omega-3 has numerous health benefits, including slowing the ageing process. According to these findings, omega-3 fatty acids can promote healthy ageing, enhance cognitive function and protect against age-related diseases. This makes them important dietary components for individuals who want to maintain their health and wellbeing as they age. Despite these promising findings, further research is required. FDA-approved fatty acid prescriptions (icosapent ethyl, omega-3-acid ethyl esters, omega-3-carboxylic acids and omega-3-acid ethyl esters A) are generally safe and do not cause adverse reactions such as eructation, dyspepsia, diarrhoea, gas, nausea or arthralgia(Reference Skulas-Ray, Wilson, Harris, Brinton, Kris-Etherton and Richter115).
Coenzyme Q10
Animal cells produce the small, lipid-soluble antioxidant molecule coenzyme Q, widely located in cell membranes(Reference Laredj, Licitra and Puccio116). Mevalonic acid and phenylalanine are the main sources of CoQ10. In a healthy individual, synthesis of CoQ10 occurs in all tissues. The synthesis of CoQ10 takes place through a precise sequence of enzymes, specifically complex Q, which is primarily located in the mitochondrial matrix membrane and endoplasmic reticulum. The pathway of mevalonic acid plays a crucial role in cholesterol synthesis by HMG-CoA reductase, which serves as the key regulatory step that is effectively inhibited by statins (Fig. 2). The internal biosynthesis of CoQ10 decreases during ageing, so it is imperative to obtain CoQ10 through our diet(Reference Gutierrez-Mariscal, Yubero-Serrano, Villalba and Lopez-Miranda117). CoQ10 is primarily found in animal meat and vegetables such as lamb, pork, chicken, beef and fish, as well as broccoli, spinach, peas and cauliflower, and fruits such as oranges, strawberries and apples. In addition, CoQ10 can be found in cereals such as rye and wheat(Reference Gutierrez-Mariscal, Yubero-Serrano, Villalba and Lopez-Miranda117). Notably, animal organs such as chicken legs, herring, trout and heart are excellent sources of CoQ10. Since CoQ10 has a large molecular size and low water solubility, its bioavailability is limited. The development of nanoparticle encapsulation, liposomal delivery systems and emulsified formulations has been used to enhance this effect. Encapsulating CoQ10 in nanoparticles allows the body to more readily absorb it. By encasing CoQ10 in lipid bilayers, lipid delivery enhances its solubility and transport across cell membranes. Droplets of CoQ10 are broken down into smaller, more absorbable ones in emulsified formulations. Through these technological advances, CoQ10’s bioavailability has significantly improved, which enhances its therapeutic effects(Reference Maciejewska-Stupska, Czarnecka and Szymański118). An adequate daily intake is considered to be between 3 and 5 mg. When supplemented externally, plasma CoQ10 levels increase but tissue levels do not(Reference Garrido-Maraver, Cordero, Oropesa-Avila, Vega, de la Mata and Pavon119). The presence of tissue uptake and successful treatment in various human diseases proves its efficacy(Reference Gutierrez-Mariscal, Yubero-Serrano, Villalba and Lopez-Miranda117,Reference Zozina, Covantev, Goroshko, Krasnykh and Kukes120) . The function of CoQ10 is to facilitate the transfer of electrons between complexes I/II and III in the electron transport chain(Reference Gutierrez-Mariscal, Yubero-Serrano, Villalba and Lopez-Miranda117). As a key component of cellular function, it is widely distributed in all cell membranes. As a component of the mitochondrial electron transport chain, ubiquinone plays a crucial role. Complex I (reduced nicotinamide adenine dinucleotide (NADH)-coenzyme Q oxidoreductase) receives electrons from donors, complex II (succinate dehydrogenase) consists of flavin-linked dehydrogenases, which oxidise fatty acids and branched-chain amino acids, and electron transfer factor Q oxidoreductase (ETF-QO) transfers electrons to complex III (ubiquinone–cytochrome C oxidoreductase)(Reference Lenaz, Fato, Di Bernardo, Jarreta, Costa and Genova121,Reference Olson and Rudney122) . CoQ10 exists in three chemical forms: semiquinone, ubiquinone and ubiquinol. The proton-motive Q cycle within mitochondrial membranes generates a proton motive force for ATP production(Reference Alcázar-Fabra, Navas and Brea-Calvo123,Reference Lenaz, Fato, Castelluccio, Genova, Bovina and Estornell124) . Various studies have demonstrated that CoQ10 supplementation exerts epigenetic effects on genes associated with different biological processes, including intermediary metabolism, signalling, transcription control, transport and phosphorylation, disease mutation and embryonic development. The mitochondrial free radical theory of ageing asserts that damage to mitochondria is pivotal in cellular ageing(Reference Harman125). Activated mitochondrial DNA is a significant target for mitochondrially derived reactive oxygen species (ROS), according to Eirin et al.(Reference Eirin, Lerman and Lerman126). Overall survival (OS) accumulation in mitochondrial DNA may contribute to ageing. Mitochondrial DNA damage is a serious concern because it can lead to a decline in the efficiency of the respiratory chain. DNA lesions and ROS result from such damage, which, in turn, can hasten the cellular ageing process(Reference Poulose and Raju127). Therefore, it is essential to protect and maintain mitochondrial DNA integrity to prevent cellular ageing and associated health problems. By interacting directly with DNA repair enzymes, CoQ10H2 promotes efficient repair of oxidative damage(Reference Schniertshauer, Gebhard, van Beek, Nöth, Schon and Bergemann128). CoQ10 levels decline with age, and inborn errors in its synthesis can further impact these levels(Reference Mantle and Dybring129). Chronic inflammation is a common problem related to ageing. CoQ10 can reduce free radicals, decrease NF-κB activation and lower the release of pro-inflammatory cytokines such as IL-6, TNF-α and C-reactive protein (CRP)(Reference Olivieri, Lazzarini, Babini, Prattichizzo, Rippo and Tiano130). Ageing-related reduction in CoQ10 levels is a significant contributor to inflammation. A study has shown that CoQ10 supplementation is effective in reducing inflammation. CRP, IL-6 and TNF-α levels were significantly reduced by CoQ10 supplementation(Reference Fan, Feng, Chen, Qin, Fu and Chen131). CoQ10’s epigenetic effects regulate NF-κB1, thereby effectively reducing inflammation(Reference Schmelzer, Lindner, Rimbach, Niklowitz, Menke and Döring132). Cardiovascular disease is one of the common ageing-related concerns. Low levels of CoQ10 in endomyocardial tissues are strongly correlated with heart failure severity, whereas CoQ10 treatment has been shown to improve cardiac contractility(Reference Sharma, Fonarow, Butler, Ezekowitz and Felker133). Treatment with CoQ10 has been reported to improve lipid profiles, significantly contributing to the management of cardiovascular disease(Reference Jorat, Tabrizi, Mirhosseini, Lankarani, Akbari and Heydari134). Clinical studies on CoQ10 supplementation in patients with antiphospholipid syndrome have demonstrated a significant reduction in thrombotic markers and pro-inflammatory markers, as well as improved mitochondrial function and endothelial health(Reference Pérez-Sánchez, Aguirre, Ruiz-Limón, Ábalos-Aguilera, Jiménez-Gómez and Arias-de la Rosa135). These findings provide strong evidence in support of CoQ10 as an effective intervention for managing antiphospholipid syndrome. It has been proposed that chronic neuro-inflammatory changes in patients with Down syndrome may accelerate AD(Reference Wilcock and Griffin136). Changes in these factors include increased levels of IL-6 and TNF-α, as well as decreased levels of CoQ10. In addition, CoQ10 levels are positively correlated with intelligence quotients(Reference Zaki, El-Bassyouni, Tosson, Youness and Hussein137). In human studies, however, inconsistent results have been found. According to a study conducted in 2003, plasma CoQ10 levels do not correlate with ageing in older women(Reference Wolters and Hahn138). According to reports, the levels of plasma and tissue CoQ10 change over time. The pancreas and adrenal glands have the highest levels of CoQ10 by 1 year of age, and the brain, heart and lungs reach their peak levels by the age of 20. However, after peak levels, CoQ10 levels decrease over time(Reference Kalén, Appelkvist and Dallner139). Other studies have confirmed that, in the brain, CoQ10 levels are decreased(Reference Söderberg, Edlund, Kristensson and Dallner140,Reference Edlund, Söderberg, Kristensson and Dallner141) . As we age, the amount of naturally produced CoQ10 in our heart decreases, and by the age of 80, only 50% of the production remains(Reference Kalén, Appelkvist and Dallner139). Furthermore, when comparing centenarians with 76-year-old individuals, ascorbic acid and total CoQ10 levels decreased in serum. It was also found that CoQ10-binding protein prosaposin increased in response to low levels of CoQ10(Reference Nagase, Yamamoto, Matsumoto, Arai and Hirose142). CoQ10 can be used to treat a number of human disorders and pathologies. Several studies have shown that CoQ10 is a safe and effective treatment for diseases in humans. In randomised controlled human trials, a daily dose of 1200 mg of CoQ10 is the maximum recommended, although short clinical trials have used doses up to 3000 mg/d(Reference Hathcock and Shao143). Older patients with chronic conditions can benefit from this treatment, CoQ10 can serve as an important coadjutant. We describe several clinical trials using CoQ10 in Table 3. The human body naturally contains CoQ10. Generally, CoQ10 supplements are well tolerated with only minor and infrequent side effects such as stomach upset, nausea, vomiting and diarrhoea.
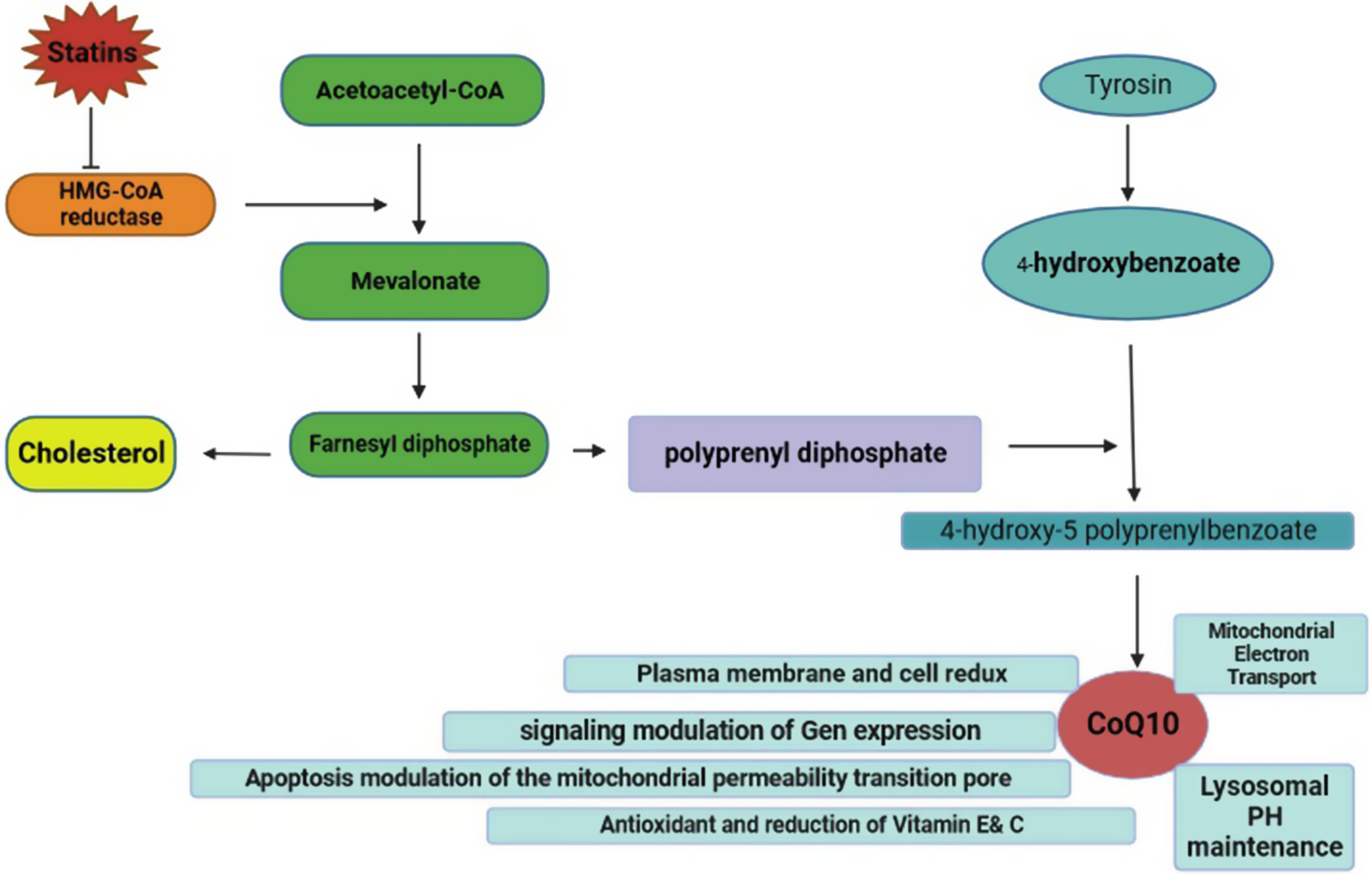
Figure 2. The potential of ginger for antioxidant and anti-inflammatory action: By translocating Nrf2 into the nucleus, it can increase the expression of Nrf2 target genes, modify Keap1 and prevent Nrf2 from proteasomal degradation. This results in an increase in GSH and a decrease in ROS. Chronic inflammation may be mediated by overexpression of COX-2 and iNOS. The ginger extract reduces inflammation by suppressing NF-κB activity through stabilising inhibitory IκBα and degrading IκBα kinase (IKK) activity. Therefore, the expression of COX-2 and iNOS is down-regulated.
Table 3. Summary of several clinical trials by CQ10
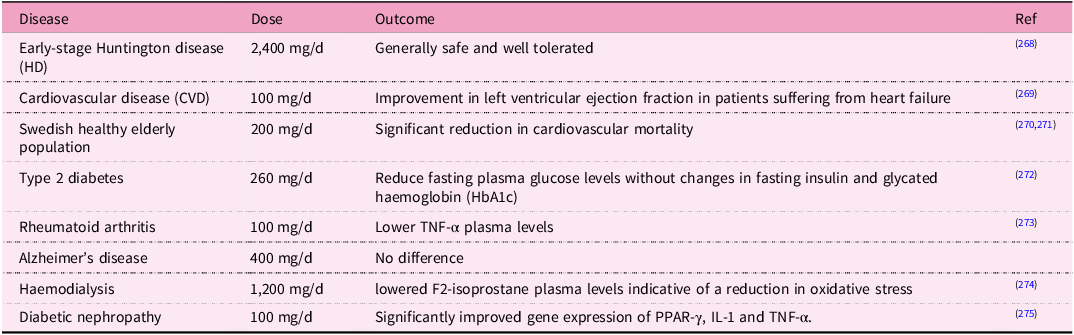
Gingerol
Originally from Asia, ginger is now found in tropical environments as a member of the Zingiberaceae family of plants(Reference Singletary144). Ginger contains various substances with biological effects, including 6-gingerol, 6-shogaol, gingerdiones, 10-gingerol, paradols, 6-dehydrogingerols, gingerdiols, 3,5-diacetoxy-6-gingerdial, 5-acetoxy-6-gingerol and 12-gingerol. Various studies have demonstrated that ginger, especially 6-gingerol, has beneficial effects on health, such as reducing oxidative stress(Reference Abolaji, Ojo, Afolabi, Arowoogun, Nwawolor and Farombi145). Ginger is vital in treating many diseases and has been used for centuries(Reference Mohd Sahardi and Makpol146). It contains various bioactive substances and components that have demonstrated different therapeutic benefits, including antibacterial, anti-inflammatory, anticancer, antidiabetic, gastroprotective, neuroprotective and antioxidant effects(Reference Mohd Sahardi and Makpol146). It can alleviate the symptoms of some illnesses, such as ulcerative colitis, Crohn’s disease, urinary tract inflammatory problems, psoriasis, lupus, rheumatoid arthritis and cancer(Reference Ballester, Cerdá, Arcusa, Marhuenda, Yamedjeu and Zafrilla147). Ginger extract could act as an antioxidant by removing excess free radicals (hydrogen peroxide and superoxide radicals). Lung disease-causing oxidative stress can be reduced by ginger, which enhances lung function. Ginger is believed to function by scavenging free radicals and modulating the inflammatory response in the lungs. Ginger can reduce inflammation by blocking the enzyme COX-2 that causes it. It can also lower the levels of IL-β and TNF-α, which are molecules that trigger inflammation. In addition, ginger contains active substances, including 6-gingerol and 6-shogaol, that can fight cancer by reducing COX-2 levels, blocking NF-κB activity in DNA and increasing BAX expression(Reference Kim, Chun, Kundu and Surh148,Reference Miyoshi, Nakamura, Ueda, Abe, Ozawa and Uchida149) . Despite its biological properties, 6-gingerol’s low bioavailability limits its application. To overcome these limitations, new encapsulation and solubilisation techniques have been introduced, including nanoemulsions, complexations, micelles and solid dispersions. As a natural antioxidant, preservative and flavour enhancer, 6-gingerol could be used to maintain food quality and shelf life by combining with various ingredients in a synergistic manner(Reference Hu, Yu, Wang, Bai, Tang and Yang150).
Ginger is a natural substance that has beneficial effects on health and longevity (Fig. 3). In addition to preventing or delaying age-related diseases, it can improve the quality of life in older adults. Ginger can also enhance the lifespan of healthy individuals by modulating cellular and molecular pathways of ageing(Reference Butt and Sultan151). Oxidative stress occurs when reactive oxygen species (ROS) overwhelm antioxidant defences in cells. Ageing and diseases associated with ageing are caused by oxidative stress. Oxidative stress and chronic inflammation cause molecular and cellular changes, ranging from genomic instability and epigenetic changes to mitochondrial dysfunction and cellular senescence(Reference Izadi, Sadri, Abdi, Serajian, Jalalei and Tahmasebi152). Physiological functions decline with ageing owing to the accumulation of oxidative damage, a concept known as the free radical ageing theory(Reference Ozkur, Benlier, Takan, Vasileiou, Georgakilas and Pavlopoulou153). Both C. elegans and mammals are believed to be affected by oxidative stress according to the free radical theory of ageing. ROS levels determine the organism’s lifespan(Reference Arulkumar, Bang, Noh, Yokozawa and Chung154), and free radicals are a major contributor to ageing progression(Reference Ziada, Smith and Côté155). ROS levels increase as a result of infection, inflammation, stress and exposure to substances such as NO x pollutants, tobacco smoke, radiation, drugs (for example, acetaminophen) and sunlight(Reference Checa and Aran156). Based on new findings, some natural products can prevent, reduce and treat ageing and diseases related to ageing. It is suggested that ginger modifies molecular targets of the pathogenesis of age-related diseases(Reference Ozkur, Benlier, Takan, Vasileiou, Georgakilas and Pavlopoulou153). There is evidence that ginger can fight inflammation and delay the ageing process in different organs, according to many studies(Reference Ilkhanizadeh, Shirpoor, Khadem Ansari, Nemati and Rasmi157). Ginger possesses strong antioxidant properties, helping to neutralise ROS and maintain a balance, known as redox balance, between ROS and antioxidants in the body. A high consumption of antioxidants can result in reductive stress. Nevertheless, excessive ginger consumption or improper use could disrupt this balance by overstimulating antioxidant defences or influencing oxidative stress-related pathways in the body. If ROS levels are not adequately controlled, this imbalance can cause oxidative damage. However, ginger is generally considered beneficial for its antioxidant properties when consumed moderately(Reference Shaukat, Nazir and Fallico158). Studies have shown that ginger extract can lower the body’s ROS and malondialdehyde (MDA). MDA is a lipid peroxidation marker harmful to cells(Reference Romero, Forero, Sequeda-Castañeda, Grismaldo, Iglesias and Celis-Zambrano159). glutathione peroxidase (GPx) activity was increased in diabetic rats after ginger treatment, suggesting that antioxidant enzymes are recovering. In addition to lowering caspase-3 activation and the Bax/Bcl-2 ratio, ginger extract also inhibited IL-1 production(Reference Shanmugam, Mallikarjuna, Nishanth, Kuo and Reddy160,Reference Thomas, Fuller, Whittles and Sharif161) . By blocking the activation of caspase-3, ginger extract may prevent apoptotic signalling that contributes to disease development(Reference Asl, Pourheydar, Dabaghian, Nezhadi, Roointan and Mehdizadeh162). The level of BDNF, a key molecule for neuronal health, function, learning and memory, was increased in the brains of mice with Scopolamine (SCO)-induced cognitive impairment after they received oral ginger extract(Reference Kim, Seo, Lee, Park and Jang163). Ginger extract administration resulted in less oxidative stress in the liver tissue of mice, with enhanced levels of antioxidant enzymes such as superoxide dismutase (SOD), glutathione reductase (GR), glutathione peroxidase (GPx) and catalase (CAT)(Reference Al Syaad, Elsaid, Abdraboh and Al-Doaiss164).

Figure 3. Function and metabolic pathway for the biosynthesis of CoQ10. The mevalonic acid pathway is responsible for cholesterol and coenzyme Q10 biosynthesis. Statins are drugs that inhibit the enzyme hydroxy-methylglutaryl-coenzyme A (HMG-CoA) reductase. This interference prevents the conversion of HMG-CoA to mevalonate, which in turn blocks the production of farnesyl pyrophosphate (PP). Farnesyl pyrophosphate is an intermediate in synthesising coenzyme Q10 and other vital compounds. The main function of coenzyme Q10 is represented.
One of the hallmarks of ageing is a persistent pro-inflammatory state. This condition, also known as ‘inflammaging’, involves chronic low-level inflammation. Many diseases affecting older adults are linked to chronic inflammation, including hypertension, atherosclerosis, diabetes and cancer(Reference Baechle, Chen, Makhijani, Winer, Furman and Winer165). Higher amounts of inflammatory molecules, such as TNF-α and IL-6, were detected in older people. These molecules can cause tissue damage and accelerate ageing(Reference Rea, Gibson, McGilligan, McNerlan, Alexander and Ross166). Inflammatory mediators such as prostaglandins and leukotrienes are blocked by ginger’s anti-inflammatory properties, which include inhibiting their synthesis. Prostaglandin E2 (PGE2), a pro-inflammatory molecule, is suppressed by both fresh and dried ginger extracts when activated by lipopolysaccharide, a bacterial toxin. The results indicated that ginger extract lowered the levels of PGE2, MCP-1, TNF-α, MPO and IL-6, which are inflammatory mediators. The extract of ginger mainly exerted its anti-inflammatory effect by preventing the migration and activation of inflammatory cells(Reference Ezzat, Ezzat, Okba, Menze and Abdel-Naim167). Ginger also has anti-inflammatory properties by reducing the breakdown of IκBα and preventing the movement of p65 into the nucleus, where it activates NF-κB(Reference Mao, Xu, Cao, Gan, Corke and Beta168).
Genomic damage and instability result from the interrelated pathological processes of chronic inflammation and oxidative stress. The biomarker γ-H2AX, which is sensitive and reliable, revealed that 6-gingerol (6-GN) treatment increases DNA damage significantly. P53 is reactivated by intracellular ROS. Due to this, p53 arrests cell cycle progression during G2/M(Reference Rastogi, Gara, Trivedi, Singh, Dixit and Maurya169). Compared with other supplements, ginger exhibited remarkable activity in protecting DNA at different concentration levels and had the highest overall antioxidant activity(Reference Deorukhkar, Ahuja, Mercado, Diagaradjane, Raju and Patel170). In breast cancer cells, 6-SG inhibited NOTCH signalling and induced apoptosis and autophagy(Reference Bawadood, Al-Abbasi, Anwar, El-Halawany and Al-Abd171). The human pancreatic PANC-1 cancer cell line is affected by ginger extract, which inhibits its cell cycle progression and triggers its apoptotic cell death. One possible mechanism by which ginger inhibits cancer growth is by generating ROS in tumour cells(Reference Akimoto, Iizuka, Kanematsu, Yoshida and Takenaga172). 6-GN inhibits the expression of AKT, NF-ĸB and Bcl2 and upregulates TNFα, BAX, cytochrome c, PARP and caspase-3. 6-GN triggers cancer cell death, possibly through caspase-3-dependent apoptosis and autophagy(Reference Chakraborty, Bishayee, Ghosh, Biswas, Mandal and Khuda-Bukhsh173). 6-GN has been shown to reduce inflammation by lowering the levels of IL-1β, TNF-α, COX-2 and iNOS, and to suppress cell growth by enhancing the expression of β-catenin, DVL-2, and WNT3a proteins and, by contrast, diminishing the expression of Ki-67 and cyclin D1 proteins(Reference Farombi, Ajayi and Adedara174).
Ginger has demonstrated cognitive benefits in older subjects. Taking 400 or 800 mg of ginger daily for 2 months improved attention and cognitive processing in middle-aged women without adverse effects(Reference Saenghong, Wattanathorn, Muchimapura, Tongun, Piyavhatkul and Banchonglikitkul175). Cholinesterase breaks down acetylcholine through the action of the active compounds in ginger. Acetylcholine is a neurotransmitter that plays a crucial role in learning and memory processes. As a result, ginger increases acetylcholine levels, thereby enhancing cognitive functions(Reference Ghayur, Gilani, Ahmed, Khalid, Nawaz and Agbedahunsi176).
The prevalence of neurodegenerative diseases increases with ageing, with AD being a common form of age-related cognitive decline that affects brain structure and function. Ginger extract enhanced the expression of CAT and SOD enzymes in the brain and reduced the secretion and expression of IL-1β , NF-ĸB and MDA levels, improving behavioural dysfunction(Reference Zeng, Zhang, Lu, Xiao, Zong and He177). Study results show that supplementing middle-aged women with ginger extract at 400–800 mg/d improved their cognitive function(Reference Saenghong, Wattanathorn, Muchimapura, Tongun, Piyavhatkul and Banchonglikitkul175). One main factor that increases the likelihood of developing cardiovascular diseases is ageing. A possible way to reduce blood lipids and blood pressure and inhibit platelet clumping is by using ginger and some of its compounds that have these effects. The blood pressure of rats under anaesthesia decreased after they received fresh ginger extract through their veins. The extract seemed to block voltage-dependent calcium channels. Ginger supplementation of 3 g/d for 45 d significantly lowered serum cholesterol and triacylglycerol in patients with hyperlipidaemia(Reference Zhao and Chen178). 6-Gingerol inhibited the activation of p38 MAPK, a mitogen-activated protein kinase involved in cardiac remodelling(Reference Lai, de Oliveira Otto, Lemaitre, McKnight, Song and King103). In addition, 6-gingerol may reduce the accumulation of fat in the liver with age(Reference Li, Wang, Yao, Ma, Chen and Han179).
Sarcopenia is a condition that affects older people and causes a loss in muscle mass, muscle size and muscle function. This can lead to problems with mobility, balance and strength. In addition to rejuvenating muscle cells, ginger’s antioxidant and anti-inflammatory properties may help prevent and treat sarcopenia(Reference Khezri, Maleki Dizaj, Rahbar Saadat, Sharifi, Shahi and Ahmadian180). Taking ginger supplements may also benefit knee osteoarthritis, a common condition in older adults, by reducing pain and improving joint function(Reference Mashhadi, Ghiasvand, Askari, Hariri, Darvishi and Mofid181). One of the most noticeable effects of ageing is the alteration of the skin’s appearance and structure. Using a body cream containing ginger oil for a month diminished signs of skin ageing, possibly due to the plant’s ability to scavenge free radicals(Reference Leelapornpisid, Wickett, Chansakaow and Wongwattananukul182). Ginger has many health benefits that may support healthy ageing and longevity. Gingerol is a compound that can improve body function and prevent ageing-related diseases. Therefore, ginger may be a natural way to support healthy ageing and longevity. According to the US Food and Drug Administration, ginger root is safe in amounts up to 4 g/d. Higher doses, however, can result in gastrointestinal discomfort, allergic reactions, prolonged preexisting bleeding, depression of the central nervous system, and arrhythmias. Ginger root consumption of more than 6 g can exacerbate gastrointestinal disturbances such as heartburn, gastrointestinal reflux and diarrhoea. A small number of cases have been reported of arrhythmia caused by this spice. Gallstone formation can be aggravated by increased bile acid secretion(Reference Ryan and Morrow183).
Curcumin
Curcumin is a potent and effective anti-ageing compound that is easily accessible and safe for use. Curcumin, a polyphenol nutraceutical, is derived from the rhizome of Curcuma longa (a member of the ginger family) and is found in turmeric(Reference Tyagi, Prasad, Yuan, Li and Aggarwal184). Curcumin is a commonly consumed spice and yellow food dye. In aqueous media, curcumin is poorly soluble and stable, leading to poor absorption by intestinal cells, with rapid metabolism by the liver. It is also quickly eliminated from the body(Reference Liu, Zhai, Heng, Che, Chen and Sun185). Due to its low bioavailability, curcumin’s therapeutic potential may be limited. Several approaches have been used to maximise curcumin absorption in the body. One such approach is to take curcumin together with piperine, the active ingredient in black pepper. Using this technique, curcumin can be more readily absorbed into the bloodstream, increasing its concentration by up to 2000%(Reference Shoba, Joy, Joseph, Majeed, Rajendran and Srinivas186). Enhanced aqueous solubility, stability and cell targeting of curcumin can be achieved by various means, including conjugation with alginate, self-assembling peptide nanofibre carriers and formulation of curcumin phospholipid complexes, microemulsions, liposomes, polymeric micelles and nanoparticles(Reference Liu, Zhai, Heng, Che, Chen and Sun185,Reference Dey and Sreenivasan187) . Meanwhile, the nanoformulation of this compound has been evaluated in various studies, and its anti-inflammatory, antioxidant and immunomodulatory effects have been proven. The form of nanocurcumin is recommended as a popular and effective form owing to its high stability in the body environment, long half-life and high bioavailability and easier access to different sites(Reference Abbaspour-Aghdam, Hazrati, Abdolmohammadi-Vahid, Tahmasebi, Mohseni and Valizadeh188–Reference Tahmasebi, Saeed, Temirgalieva, Yumashev, El-Esawi and Navashenaq190). Clinical trials have shown that high daily doses of curcumin, up to 12 g/d, are considered safe for patients(Reference Cheng, Hsu, Lin, Hsu, Ho and Shen191). Curcumin exhibits pleiotropic activity, similar to other polyphenols. It can induce cellular responses by interacting with multiple proteins. Curcumin can positively influence cellular processes by regulating microRNAs and influencing epigenetic changes in cells(Reference Gupta, Kismali and Aggarwal192). As we age, persistent low-grade inflammation often occurs(Reference Calder, Bosco, Bourdet-Sicard, Capuron, Delzenne and Doré193). There is no doubt that polyphenol-rich foods can effectively alleviate symptoms of ageing owing to their potent anti-inflammatory and antioxidant properties. There is substantial evidence that curcumin has anti-ageing properties (Fig. 4)(Reference Sikora, Scapagnini and Barbagallo194,Reference Sandur, Ichikawa, Pandey, Kunnumakkara, Sung and Sethi195) . Extensive clinical trials have shown that curcumin can reduce symptoms of age-related diseases, including atherosclerosis, diabetes and cancer(Reference Olszanecki, Jawień, Gajda, Mateuszuk, Gebska and Korabiowska196,Reference He, Yue, Zheng, Zhang, Chen and Du197) . Curcumin activated SIRT1 and protected human umbilical vein endothelial cell (HUVEC) from peroxide-induced senescence(Reference Sun, Hu, Hu, Xu and Jiang198). Bis-demetoxycurcumin, a curcumin analogue, protected Wi38 fibroblasts from oxidative stress-induced senescence(Reference Kitani, Osawa and Yokozawa199). These findings suggest that curcumin and its analogues show potential for therapeutic use in combatting cellular ageing and related diseases. Curcumin’s anti-tumour activity is another important function(Reference Bielak-Zmijewska, Sikora-Polaczek, Nieznanski, Mosieniak, Kolano and Maleszewski200). Ageing is one of the most significant risk factors for tumour development(Reference Hansen201). Older adults are more susceptible to cancer than younger individuals. Half of all cancers diagnosed in older adults are prostate, lung or colon cancers. Breast, colon, lung and stomach cancers account for 48% of all cases among older women. Curcumin has been shown to protect against tumourigenesis by reducing toxicity, potentially inducing cancer cell apoptosis and inhibiting metastasis through its anti-angiogenic properties(Reference Sandur, Ichikawa, Pandey, Kunnumakkara, Sung and Sethi195). Curcumin helps slow the ageing process by targeting multiple pathways involved in age-related diseases(Reference Izadi, Sadri, Abdi, Zadeh, Jalaei and Ghazimoradi202). The pathogenesis of multiple age-related diseases involves oxidative stress-induced damage, a hallmark of ageing(Reference Luo, Mills, le Cessie, Noordam and van Heemst203). Cells and tissues that accumulate ROS can cause oxidative stress when detoxification mechanisms are overwhelmed(Reference Pizzino, Irrera, Cucinotta, Pallio, Mannino and Arcoraci204). Curcumin effectively inhibits cyclooxygenase/lipoxygenase and xanthine dehydrogenase/oxidase enzymes, significantly reducing ROS production. It boosts the activity of antioxidant enzymes SOD and POD, which serve as primary defences against free radicals. Topical application of curcumin can inhibit H2O2 production induced by tissue plasminogen activator (TPA) in the epidermis(Reference Huang, Ohigashi, Osawa, Terao, Watanabe and Yoshikawa205). Curcumin significantly enhanced the levels of antioxidant enzymes SOD and CAT, along with glutathione, in rats with diabetes(Reference Abo-Salem, Harisa, Ali, El-Sayed and Abou-Elnour206). Oyetayo et al. (2020) demonstrated that the induction of Al3+ metal ions in Drosophila melanogaster resulted in a significant decrease in antioxidants such as catalase, glutathione-S-transferase and glutathione and simultaneously caused a notable increase in free radical precursors such as H2O2 and NO. Curcumin reduced oxidative damage induced by Al3+ ions in a dose-dependent manner(Reference Oyetayo, Abolaji, Fasae and Aderibigbe207). Curcumin inhibits the production of inflammatory mediators by regulating signalling pathways. Specifically, curcumin targets Toll-like receptor-4 (TLR-4), influencing inflammation modulation. It contributes to the treatment of inflammatory diseases by regulating downstream signalling pathways, including NF-κB, mitogen-activated protein kinases (MAPK) and activator protein 1 (AP-1)(Reference Zhang, Zheng, Luo, Du, Zhang and Fu208). NF-κB, a pivotal transcription factor in inflammation, orchestrates the expression of various pro-inflammatory cytokines such as TNF-α, IL-1β and IL-6 (Reference Tahmasebi, Alimohammadi, Khorasani, Rezaei and Rezaei209). Moreover, it promotes the production of critical proteins such as TNF-α, activating NF-κB, particularly in chronic inflammation. NF-κB regulates intracellular immunity in ageing and age-related diseases(Reference Salminen, Huuskonen, Ojala, Kauppinen, Kaarniranta and Suuronen210). In addition, elevated levels of MCP-1 in circulation have been identified as a reliable biomarker for ageing, indicating that, as individuals age, the levels of MCP-1 tend to increase, making it a valuable biomarker for age-related studies(Reference Yousefzadeh, Schafer, Noren Hooten, Atkinson, Evans and Baker211). Curcumin has been shown to inhibit MCP-1, an inflammatory mediator(Reference Kunnumakkara, Bordoloi, Padmavathi, Monisha, Roy and Prasad212). Extensive research on model organisms, ranging from yeast to mice, highlights the crucial role of autophagy in the ageing process. The Autophagy gene family is crucial for lifespan extension, and increasing the expression of specific autophagy proteins has been shown to further extend lifespan(Reference Hansen, Rubinsztein and Walker213). The key regulators of autophagy are mTOR kinase and AMPK. In some model organisms, extending lifespan and health span was achieved by inhibiting the mTOR pathway and activating the AMPK pathway(Reference Hansen, Rubinsztein and Walker213). These signalling pathways also regulate nutrient sensing, similar to the insulin/IGF-1 pathway. Studies have shown that inhibiting the insulin/IGF-1 pathway can effectively postpone ageing in animal models(Reference Brown-Borg and Bartke214). Curcumin’s impact on autophagy is indicated by its ability to modulate AMPK levels and activity while inhibiting mTOR (Reference Sikora, Bielak-Zmijewska, Mosieniak and Piwocka215,Reference Jiao, Wang, Lu, Tang, Chen and Mou216) . The dysregulation of the autophagy process is one of the leading causes of neurodegenerative diseases. The accumulation of mutated huntingtin and misfolded Aβ proteins has been established as crucial factors in the pathogenesis of Huntington’s and Alzheimer’s diseases. Through various mechanisms, curcumin is capable of effectively inducing the degradation of misfolded proteins and damaged organelles. Curcumin activates transcription factor EB (TFEB)(Reference Zhang, Wang, Xu, Lu, Jiang and Wang217), inducing lysosome(Reference Maiti, Rossignol and Dunbar218) biogenesis and restoring HSP70 levels for proper cargo loading. In addition, curcumin triggers the process of mitophagy(Reference de Oliveira, Jardim, Setzer, Nabavi and Nabavi219), which reduces oxidative stress levels, leading to an improvement in the survival of neurons(Reference Giordano, Darley-Usmar and Zhang220). Recent randomised clinical trials show curcumin’s positive impact on ageing-related disorders. Osteoarthritis (OA) is a chronic joint condition strongly associated with both chronic and acute inflammation(Reference Hunter, Schofield and Callander221). Curcumin has been shown to have anti-arthritic effects in humans with OA and rheumatoid arthritis(Reference Henrotin, Priem and Mobasheri222,Reference Belcaro, Hosoi, Pellegrini, Appendino, Ippolito and Ricci223) . Two groups of forty participants with mild-to-moderate knee osteoarthritis were randomised over 6 weeks. A 500 mg dose of curcuminoid was given to one group (supplemented with 5 mg of piperine per dose), while a placebo was given to the other group. In comparison with the placebo group, the treatment group showed notable reductions in the Visual analogue scales (VAS), WOMAC (It measures five items for pain (score range 0–20), two for stiffness (score range 0–8), and 17 for functional limitation) and Lequesne’s pain functional index (LPFI) scores. The pain and physical function scores based on the WOMAC subscales were significantly improved (p-value < 0·001), but stiffness scores did not show significant improvements(Reference Panahi, Rahimnia, Sharafi, Alishiri, Saburi and Sahebkar224). Curcumin supplementation has been studied in several randomised controlled trials over a 4-week period. In particular, the studies assessed GPx in erythrocytes, MDA concentrations and SOD activity. Supplementing with curcumin at daily doses of 600 mg effectively decreases circulating MDA levels and enhances SOD activity, according to these studies(Reference Qin, Huang, Gong, Shen, Huang and Tang225). The attenuation of systemic inflammation by curcumin has implications for numerous conditions affecting various systems beyond arthritis. Metabolic syndrome (MetS) is characterised by insulin resistance, hyperglycaemia, hypertension, low HDL-cholesterol, high LDL-cholesterol, elevated triacylglycerol levels and visceral obesity. It has been shown that curcumin improves insulin sensitivity(Reference Na, Li, Pan, Zhou, Sun and Meng226), suppresses adipogenesis(Reference Bradford227) and reduces elevated blood pressure(Reference Hlavačková, Janegová, Uličná, Janega, Cerná and Babál228), inflammation(Reference Sahebkar229) and oxidative stress associated with MetS(Reference Ak and Gülçin230). Thirty-four patients with AD were studied in a randomised, double-blind, placebo-controlled study by Baum et al. There were two different doses of curcumin (1 or 4 g) and a placebo group receiving 4 g. The Mini-Mental State Examination (MMSE) score did not improve after curcumin treatment(Reference Baum, Lam, Cheung, Kwok, Lui and Tsoh231). Curcumin delays disease progression and modulates cognitive function as well as biomarker levels. Clinical trials may have been unsuccessful due to curcumin’s poor bioavailability, the selection of cohorts at an advanced stage of AD and biological differences between rodent models and patients with AD. Many interventions have failed in clinical trials despite their success in animal models(Reference Chen, Du, Zheng, Li, Zhou and Zhang232). Curcumin’s safety and efficacy have been demonstrated in numerous clinical trials involving healthy individuals. However, it has been associated with some adverse effects. Researchers showed that side effects such as diarrhoea, headache, rash and yellow stools were reported in a dose–response study using 500–12 000 mg of curcumin over 72 h. Another study noted nausea, diarrhoea and elevated levels of serum alkaline phosphatase and lactate dehydrogenase following daily doses ranging from 0·45 to 3·6 g/d(Reference Hewlings and Kalman233).
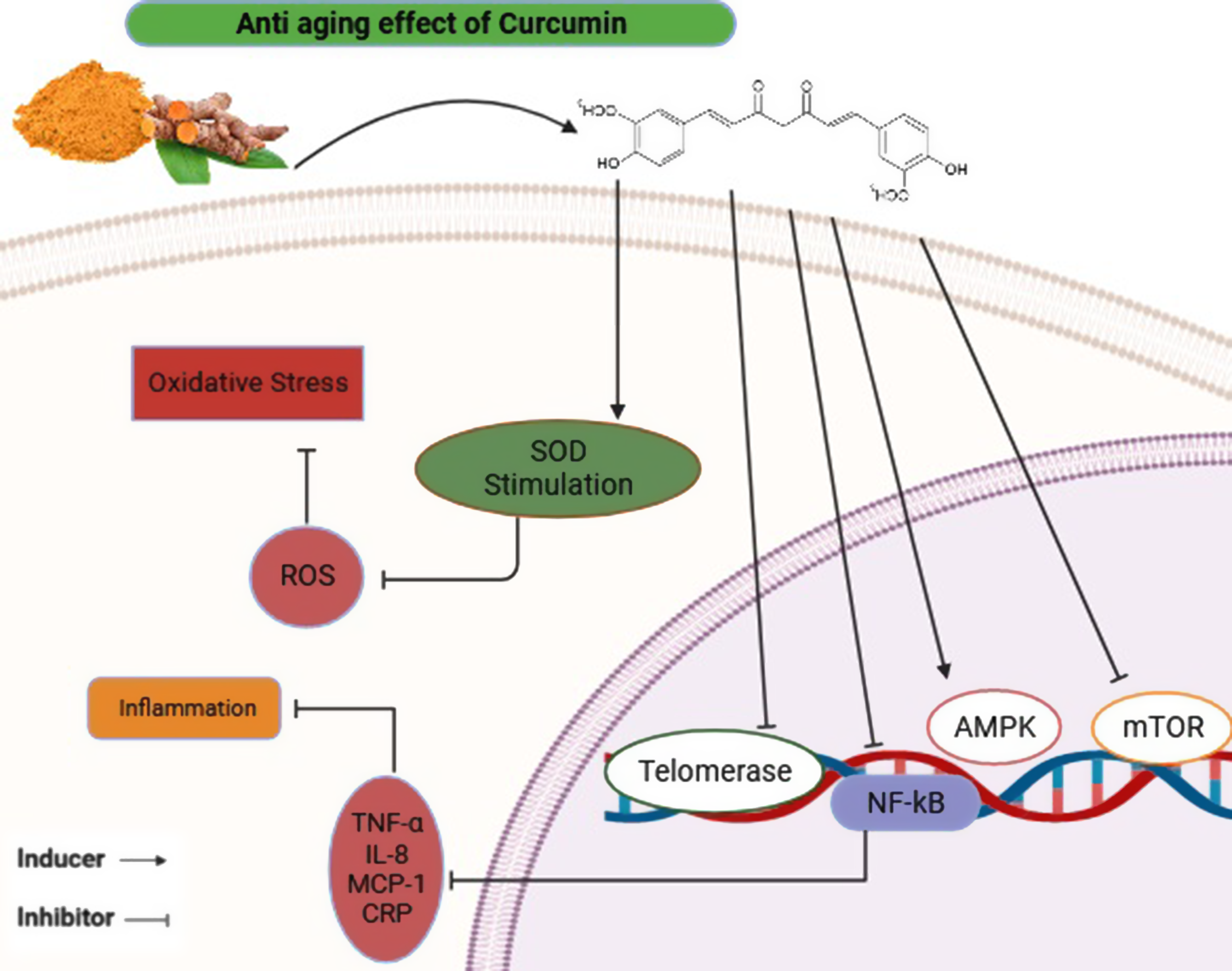
Figure 4. Anti-ageing effects of curcumin. Curcumin is a substance that has been found to have broad anti-ageing effects. At the cellular level, it can increase the level or activity of anti-ageing factors such as AMPK while suppressing pro-ageing factors such as NF-κB, mTOR and telomerase. Curcumin can also help to reduce oxidative stress by decreasing ROS levels. In addition, it can modulate inflammation by inhibiting or reducing the levels of anti-inflammatory cytokines, which can help to delay the ageing process.
Potential synergistic effects of supplements
In addition to their complementary roles in enhancing health, omega-3 fatty acids, coenzyme Q10, gingerol and curcumin may have synergistic effects. The combined effects of omega-3 fatty acids and coenzyme Q10 might further improve cardiovascular health and energy metabolism. A study confirmed that omega-3 and coenzyme Q10 combined significantly prevented the progression of hypercholesterolaemia-induced atherosclerosis. This study investigated the effects of omega-3 and/or coenzyme Q10 (CoQ10) on hypercholesterolaemia-induced atherosclerosis in rats. The study assessed lipid profiles, cardiovascular risk indices, serum levels of adiponectin and creatine kinase, oxidative stress markers and histopathological changes in the heart and aorta. Results showed that hypercholesterolaemic rats had elevated lipid levels and oxidative stress, along with decreased adiponectin levels and increased levels of creatine kinase. Treatment with omega-3 and/or CoQ10 improved lipid profiles, reduced oxidative stress and ameliorated histopathological damage. The combination of omega-3 and coenzyme Q10 provided the most significant benefits, suggesting their effective anti-hyperlipidaemic, cardioprotective and atheroprotective effects, supporting their use in managing cardiovascular disorders in obese individuals(Reference Ibrahim Fouad234). A synergistic approach to combination therapy is emerging as a method for treating complex diseases, including inflammation(Reference Xiao, Zhang, Viennois, Kang, Zhang and Han235). Turmeric and ginger are known to exert anti-inflammatory actions through many common signalling pathways, including Nrf2 activation(Reference Muhammad, Wang, Li, Li and Zhang236,Reference Chen, Tang, Sun, Veeraraghavan, Mohan and Cui237) . Gingerol and curcumin have antioxidant and anti-inflammatory properties, which may help reduce both inflammation and oxidative stress. When combined, these compounds might provide a more robust defence against age-related conditions(Reference Zhou, Afzal, Wohlmuth, Münch, Leach and Low238–Reference Bakhtiari Aqmasjed, Sajjadi, Falahatkar and Safari240). One study evaluated the combined analgesic, anti-inflammatory and haemolytic effects of ethanolic extracts from turmeric (Curcuma longa) and ginger (Zingiber officinale) through in vitro and in vivo experiments using albino mice and Wistar rats. Results showed that all doses significantly reduced inflammation within 1–4 h, with the combination of ginger (200 mg/kg) and turmeric (60 mg/kg) showing the greatest inhibition at the 4-h mark(241). Another study investigated the effects of curcumin and long-chain omega-3 polyunsaturated fatty acids (LCn-3PUFA) supplementation on glycaemic control and blood lipid levels in individuals at high risk for type 2 diabetes (T2D). In a 12-week, double-blinded, placebo-controlled trial with sixty-four participants, subjects were randomly assigned to four groups: double placebo (PL), curcumin plus placebo (CC), LCn-3PUFA plus placebo (FO) or a combination of curcumin and LCn-3PUFA (CC-FO). The primary outcomes included HbA1c, fasting glucose and insulin sensitivity. Results showed that, while HbA1c and fasting glucose levels did not change significantly, insulin sensitivity improved significantly in the CC group compared with PL. FO and CC-FO also improved insulin sensitivity, though not significantly. Triacylglycerol levels increased in the PL group but decreased with CC and CC-FO supplementation, with FO showing the greatest reduction. The study concludes that, while curcumin and LCn-3PUFA supplementation may reduce insulin resistance and triacylglycerol, they did not show complementary benefits for glycaemic control(Reference Thota, Acharya and Garg242). Research into their combined effects is still evolving, but preliminary findings suggest that this synergy could contribute to more effective strategies for promoting overall health and combatting age-related conditions.
Concluding remarks
Various factors, primarily environmental and genetic, influence the process of ageing. In recent decades, molecular studies have contributed to our understanding of ageing mechanisms, particularly genes such as sir-2·1, ctl-1, daf-16 and sod-3. In addition, oxidative enzymes have been optimised to perform anti-ageing functions, such as enhanced expression of FOXO3a and Akt proteins, along with increased activity of SOD and CAT. Although our understanding of the biological mechanisms underlying ageing has made significant progress, preventing and delaying ageing-related diseases remains a challenge. In recent years, anti-ageing agents derived from natural active ingredients have been extensively studied. They offer significant benefits to patients by improving quality of life and extending survival. These agents not only eliminate free radicals, inhibit inflammation and enhance the activity of various oxidative enzymes but also arrest the cell cycle, regulate oncogenes, prevent diabetes, reduce adipocyte formation and interact with multiple signalling pathways involved in disease pathogenesis. In addition, older adults often consume these natural dietary supplements, indicating they may help promote healthy ageing and reduce the adverse effects associated with ageing. In this study, nutrient-sensing pathways are emphasised as a potential pathway to extend longevity by natural supplements by treating ageing-related diseases. A growing burden of ageing-related diseases presents a major global health challenge, and natural supplements may help treat these conditions. In addition to providing a theoretical background for the use of natural products as potential anti-ageing agents, this study also provides several practical applications. Several recently identified natural supplements with potential anti-ageing properties are identified in the study, which summarises the current understanding of nutrient-sensing pathways involved in the longevity-extending effects of natural supplements. Numerous animal models and clinical studies have showcased the positive effects of omega-3 fatty acids, coenzyme Q10, gingerol and curcumin in slowing down the ageing process. We identified several key research gaps that warrant further investigation. First, although these supplements have shown potential in mitigating age-related conditions, the long-term effects and optimal dosages for sustained health benefits in humans remain unclear. In addition, the interactions between these compounds and other medications commonly taken by older adults have not been thoroughly studied. Another critical gap is the need for more large-scale, randomised clinical trials that explore the effects of these compounds on specific ageing-related conditions such as neurodegenerative diseases and cardiovascular decline. However, further research is essential to unravel the mechanisms of action of these agents and assess their suitability for clinical application.
Availability of data and material
Not applicable.
Acknowledgements
Our sincere gratitude goes to authors who contributed their time and expertise to completing this article.
Authorship
Mehran Izadi, Nariman Sadri and Amirhossein Abdi contributed to hypothesis, data gathering and writing the main text of the manuscript. Mohammad Mahdi Raeis Zadeh, Mohammad Mahdi Ghazimoradi, Sara Shouri and Dorsa Jalaei contributed to the hypothesis, data gathering, designing of figure and tables, and final editing. Safa Tahmasebi contributed to the writing, scientific and structural editing, hypothesis, correspondence and verifying the manuscript before submission.
Financial support
This research received no grant from any funding agency, commercial or not-for-profit sectors.
Competing interests
The authors declare that they have no competing interests.
Ethical standards
Not applicable.
Consent for publication
Not applicable.