Appendix A Radio Astronomy
Radio Waves
This is a book in which radio telescopes play a central role. While we think it is likely that most readers of our book are familiar with that subject, we would also guess that a minority of our audience (and most of the general population) do not know all that much about radio telescopes and the scientific revelations they have produced. Our aim here is to remedy this situation for our non-astronomer readers as briefly and non-technically as possible.
All astronomical telescopes share the same basic functional structure. Radio telescopes image celestial phenomena just as optical telescopes, but they do so in radio waves rather than visible light. Humans are simply not equipped to see radio waves. You can look through even a small telescope and see the mountains and craters of the Moon, but without complicated equipment, it is not so easy to detect even the strongest radio waves that reach Earth from interstellar space. Nonetheless, the underlying commonality of all telescopes is the result of the fact that the light by which we see turns out to be part of a fundamental and far grander phenomenon that is largely invisible to the eye, namely electromagnetic waves.
Visible light is an electromagnetic wave. The different colors of the light seen in a rainbow, are part of the same phenomenon, simply made up of light of slightly different wavelengths. More important, theory does not forbid electromagnetic waves of radically different wavelengths, ranging from the ultrashort (what we now call x-rays and gamma rays) to the comparatively long (what we now call the radio regime). Figure A.1 illustrates the bands of the electromagnetic spectrum.

Figure A.1 The electromagnetic spectrum from gamma rays to radio waves. Visible light occupies only a narrow slice compared with the radio band.
The characteristics of any wave are its wavelength (distance between successive wave peaks), its frequency (the number of waves passing a fixed point per second), and its speed. By definition, wavelength times frequency is equal to speed. The speed (in a vacuum) of electromagnetic waves, produced by accelerated charges, is the speed of light. The modern term “radio” usually includes all electromagnetic waves longer than the infrared. This is actually a huge span of wavelengths, running from about 100 km (approximately 60 miles) to around 1,000 millionths of a meter (1,000 microns, or about 1/25th of an inch). Obviously, dealing with such a large range of numbers can become very cumbersome, and for convenience, it is usual for scientists and engineers to subdivide the radio regime into smaller domains. Electromagnetic signals with wavelengths shorter than about 10 cm are commonly called microwaves, waves shorter than 1 cm are called millimeter waves, and waves shorter than 1 mm submillimeter waves.
Radio telescopes observe four types of cosmic radiation: synchrotron, bremsstrahlung, thermal, and spectral line. Figure A.2 illustrates the first of these mechanisms. Synchrotron radiation is produced by free electrons following spiral paths around magnetic field lines. The electrons are constantly accelerated while following these paths and they emit electromagnetic radiation which is strongest at low frequencies in the radio band. Radio astronomy began with the observation of synchrotron radiation. It was the discovery of such radiation from the center of our Galaxy by Karl Jansky in 1933 that marks the birth of radio astronomy. Figure A.3 is an image of synchrotron emission captured by the Very Large Array (VLA) of the radio galaxy Hercules A.

Figure A.2 Synchrotron radiation produced by an electron spiraling in a magnetic field.
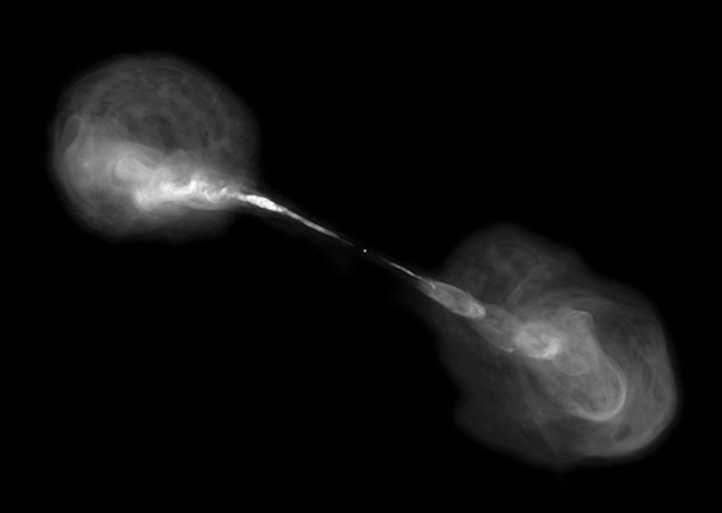
Figure A.3 The radio galaxy Hercules A as imaged by the VLA. The narrow jets emanate from a black hole in the center of the galaxy and end in the large lobes. The synchrotron emission of the radio image reveals a structure not seen in visible light; the optical emission from the galaxy itself would only show a small dot in the center.
Bremsstrahlung radiation is emitted from a plasma of ionized (largely) hydrogen atoms. The electrons are deflected in collisions with the protons, emitting radio waves. The process is illustrated in Figure A.4. The plasma is created in the interstellar medium by nearby hot, luminous stars. The Orion Nebula is a prominent example in our Milky Way Galaxy, shown in Figure A.5.

Figure A.4 An electron deflected by an ionized atom radiates bremsstrahlung radiation.

Figure A.5 The Orion Nebula imaged in bremsstrahlung radiation by the VLA. Such radiation implies a plasma of ionized atoms in this star-forming region.
Thermal radiation is produced by the random motions of electrons in a warm object; that is, any object with a temperature above absolute zero. All the room temperature objects in our daily environment, including our bodies, radiate in the infrared. At the higher temperatures found, for example, in flames, the radiation is visible; we see the glow of heated objects. Stars have temperatures that cause them to radiate in the visible light band and in the adjoining infrared and ultraviolet bands. The light thus recorded by an optical telescope is almost always starlight, and objects in the Solar System are seen in reflected light from the Sun. Images of our Galaxy and external galaxies are composed of a myriad of stars, as Galileo found when he turned his telescope to the Milky Way. In contrast, the cosmic background radiation, cool in temperature, is strongest at millimeter wavelengths. It is thermal radiation from the Big Bang. Thermal radiation from the dust in protoplanetary disks is seen in the images made by ALMA (see Chapter 10, Figures 10.1 and 10.2). ALMA, the subject of this book, is an instrument exquisitely designed for observations of thermal radiation to study the “cold universe.”
Spectral line emission occurs because the energy of atoms and molecules is confined to particular states or energy levels. For example, the tumbling motion of the carbon monoxide (CO) molecule cannot proceed at an arbitrary rate but must occur in quantum states labeled by a number that indicates their spin rate. Figure A.6 illustrates the source of three lowest frequency spectral lines of CO, namely transitions from the J = 1, 2, and 3 energy states to the next lowest energy state. Figure A.7 is an image1 of the galaxy M51 in the radiation from the J = 1 to J = 0 quantum state of CO.

Figure A.6 The three lowest frequency transitions between the quantized energy levels of CO: J = 1–0 at 115 GHz, J = 2–1 at 230 GHz, and J = 3–2 at 345 GHz. These frequencies of CO are the workhorse observational frequencies for ALMA, among the thousands of frequencies from the two hundred plus interstellar molecules.

Figure A.7 M51, the Whirlpool Galaxy, imaged in the radiation from the lowest frequency spectral line of carbon monoxide, CO, at 115 GHz. The image combines data obtained by CARMA and the Nobeyama 45 m Telescope.
Telescopes
In 1609, Galileo Galilei, who held the Chair of Mathematics at the University of Padua and who was helping to move the science of physics out of the swamp of Greek philosophy and into the realm of verifiable natural law, fabricated a small telescope. Galileo did not invent the telescope.2 However, he was, as far as is known, the first human being to turn a telescope on the night sky, explore what he found in a systematic way, and analyze and publish his findings. Galileo’s first telescope only magnified by a factor of three, but just a few months later he had built a 20-power instrument. Observations with it revealed the Moon to be a world unto itself, with mountains, craters, and what seemed to be (but were not) seas and oceans, and led to the discovery of the four largest moons of Jupiter; other observations showed that the planet Venus exhibits phases like those of the Moon, and that what appeared to the naked eye to be diffuse nebular patches of light in the Milky Way could be at least partially resolved into stars using his telescope. Galileo continued to improve his designs, and by 1611 further observations showed the Sun was not the perfect orb presumed by philosophers; instead, it had a surface harboring small, dark spots that revealed a slow rotation about its axis.
For the next 320 years, every astronomical telescope built on Earth was essentially an elaboration of Galileo’s first instrument, designed with one fundamental goal: to extend the reach of human vision. Beginning in the early twentieth century, astronomers began using new technologies to build instruments aimed at portions of the electromagnetic spectrum that lay beyond the narrow range of wavelengths directly visible to the human eye, and by the latter part of the twentieth century, astronomers had added gamma-ray, x-ray, ultraviolet, infrared, and radio telescopes to their kit.
Nevertheless, most modern radio telescopes share a common basic architecture: a large collecting element to gather and focus faint light from the sky. This is followed by a “receiving system” which detects and amplifies the light, digitizing and recording an image of the observations. This latter process is directly comparable to the way the lens, electronic sensor, and memory card of modern digital cameras operate to produce a digital image.
The generic structure of a typical radio telescope is quite similar to that of optical reflecting telescopes. Notably, there is a signal collecting element – usually a reflecting surface – that is functionally identical to the main mirror and subsidiary light-handling elements of an optical telescope. Radio waves that are captured by the reflector are then focused to go through a sequence of electronic systems that detect, amplify, digitize and finally record the captured signals, just as in the case of modern optical telescopes and digital cameras. Radio astronomers still use the descriptive term “receiver” for the electronics responsible for the initial stage of signal detection. An important common feature of most optical and radio telescopes arises because both types of instruments must have the ability to continuously follow astronomical sources across the sky in order to compensate for their apparent motion caused by the Earth’s rotation. Figure A.8 shows the functional outline of a single-dish radio telescope.

Figure A.8 Conceptual diagram of a single-dish radio telescope.
While single-dish radio telescopes typically have a close one-to-one correspondence with their optical counterparts functionally, sometimes more specialized radio telescope designs are required to overcome technical or physical performance limitations. Invariably, such designs will involve tradeoffs. We discuss one of these next.
Radio Interferometers
A particularly acute performance limitation of single-dish radio telescopes is their relatively poor resolution. The term resolution here simply means the detail that can be seen in an image. It is measured as the angle subtended by two barely discernable objects as seen by the observer. The resolution of any telescope that is not limited by atmospheric distortion is proportional to the wavelength of the electromagnetic waves it is designed to detect divided by the size of its main reflector. Since radio waves are defined by having enormously larger wavelengths than visible light waves, this means that a radio telescope must be enormously larger than any given optical telescope if it is to provide an image of comparable detail. Consequently, a single-dish radio telescope operating at a wavelength of 1 mm would have to be nearly 3 km in diameter if one wanted to produce radio images with the same level of detail as the Hubble Space Telescope.
Building a steerable, rigid, radio telescope dish 3 km in diameter is a structurally hopeless task. An antenna that size would have to be supported at its center, which would therefore have to sit on a pedestal or other support structure around 1.5 km in height. This is about twice the height of the Burj Khalifa, the tallest building yet built by the human race. More to the point, the largest steerable radio telescope in the world has a main dish around 100 m in diameter, close to the limiting size possible.3
It is this frustrating situation that led radio astronomers to develop instruments called imaging interferometers, which can provide the resolution of much larger radio telescopes by using many smaller radio antennas to at least partially fill the “footprint” of the larger antenna they are unable to build. Superficially, an imaging interferometer looks like an array of similar radio telescopes that observe the same source at the same time. This appearance masks a significant increase in complexity. In an interferometer, the individual telescope outputs are connected to a highly specialized computer, called a correlator, which combines the data streams to form an image having a resolution comparable to what would be produced by an antenna whose diameter is equal to the largest separation of the various small antennas in the array.
A price is paid for the gain in resolution. An imaging interferometer is in some ways a poor astronomer’s giant telescope, that is, one missing most of its reflecting surface. It is a “sparsely filled” aperture. While the notional giant antenna would catch all the radio waves that fall on its reflecting surface, a sparsely filled aperture of the same size can only collect signals that happen to fall on the parts of the giant antenna’s footprint that are occupied by smaller antennas. Thus, an imaging interferometer cannot match the sensitivity of the ideal giant telescope. There is also an important technical issue associated with the images produced by sparse arrays. Due to missing reflector elements relative to the ideal fully filled surface, there is irretrievable missing information needed to fully reconstruct radio images. Put another way, the images produced by radio interferometers are estimates of what a giant full aperture telescope would yield, and they require substantial off-line computer processing to attain their full potential.
NRAO’s VLA, built during the 1970s and dedicated in 1980, was the first large imaging interferometer. (Large interferometers are called arrays.) The VLA is shown in Figure A.9. Although interferometers are much more complex than single-dish telescopes, they have become indispensable instruments to modern radio astronomy. ALMA is an imaging radio interferometer, the product of a long history in the development of radio telescopes.

Figure A.9 Ten of the 27 antennas that make up the Very Large Array in New Mexico.
Misconceptions
Radio telescopes have nothing whatsoever to do with radio and television sets or their broadcasts, although all involve radio waves. But this misconception is remarkably common. A resident of Green Bank, West Virginia, once complained to the Observatory that the radio astronomers were watching her through her television set, which would have been a stunning technical achievement were it even possible. Another common misconception is that radio astronomers only listen for messages from extra-terrestrial civilizations. John Kraus, a pioneer in radio astronomy, implied just that when he named the telescope he used to search for such signals the “Big Ear.” The Search for Extra-Terrestrial Intelligence (SETI) does use radio telescopes to search for artificial signals, a sign of non-terrestrial life. In 1960, the first such search was conducted at NRAO’s Green Bank site using the Tatel Telescope, an 85 ft diameter antenna. The search was called Project Ozma4 and was conducted by NRAO staff members, led by Frank Drake. The movie Contact, based on the novel of the same name by Carl Sagan, showed the star Jodie Foster listening with headphones connected to the VLA. Radio astronomers today do not use headphones, nor do they “listen.” The popularity of the movie reinforced this notion in the general public’s concept of radio astronomy. But SETI is only a small aspect of radio astronomical activity. The vast majority of radio astronomers are studying the natural emission in radio waves from celestial objects, not searching for artificial emission transmitted by a distant civilization.
Appearances can lead to misconceptions. Satellite television and radio systems may employ ground stations that include one or more large dish-type antennas for downloading content, but these are not radio telescopes. Likewise, space agencies such as NASA and sister organizations elsewhere in the world that track and operate deep space missions send commands to their spacecraft and download scientific data using large antennas that resemble radio telescopes. Their mission and purpose is not radio astronomy. Not every antenna is a radio telescope.
Finally, some radio telescopes look nothing like a dish. This is particularly true at long radio wavelengths. A classic example is the antenna, shown in Figure A.10, that Karl Jansky used in 1932 to make his discovery of radio waves from the center of the Milky Way. This was the first detection of radio waves from a non-terrestrial source, the beginning of radio astronomy.

Figure A.10 Karl Jansky standing in front of his discovery making radio telescope.
In summary, beginning with Jansky, radio astronomy has revealed an entirely new view of the Universe, one rich in discoveries.5 ALMA is the most recent example of the power of radio telescopes to advance our understanding of cosmic phenomena. We can look forward to the era of the New Generation Very Large Array (ngVLA) and the Square Kilometer Array Observatory (SKAO), which will accomplish at lower frequencies what ALMA has done at higher frequencies.
Notes
1 Koda et al. (Reference Koda2009).
2 However, the telescope was a fairly recent invention in Newton’s time, as documented by two patent applications to the Dutch government that were never granted. By 1609, details of the simple design had been widely disseminated throughout Europe. The development of lenses and the telescope has been described by the Galileo Project. http://galileo.rice.edu/sci/instruments/telescope.html.
3 The US Naval Research Laboratory attempted to build a 600 ft diameter antenna in the late 1950s at a site in Sugar Grove, Virginia, near the NRAO Green Bank site. The project was a fiasco and it was canceled in 1962 by Defense Secretary Robert McNamara. See Kellermann, Bouten, and Brandt (Reference Kellermann, Bouton and Brandt2020) for a description of the project.
4 For a history of SETI, see Drake and Sobel (1992).
5 For an account of radio astronomy discoveries, see Kellerman and Bouton (2023).