Introduction
The recognition of an extensive deep biosphere on Earth, and the advantages of subsurface niches for putative life on other planets, have contributed to the serious consideration of deep biosphere targets in the search for evidence of life on Mars (Fisk & Giovannoni Reference Fisk and Giovannoni1999; Weiss et al. Reference Weiss, Yung and Nealson2000; Michalski et al. Reference Michalski, Cuadros, Niles, Parnell, Rogers and Wright2013). The lacustrine infills of impact craters on Mars are an especially attractive target, as they may once have been sites of elevated heat flow (driving the circulation and surface expression of liquid water) and nutrient accumulation, enhancing habitability (Arp Reference Arp1995). Sulphate minerals have been detected in numerous crater-hosted sediments thought to be groundwater-fed palaeolake deposits, where they have been interpreted as evaporites (sulphate also fills diagenetic veins on Mars; Ehlmann & Edwards Reference Ehlmann and Edwards2014 and references therein). The importance of lacustrine deposits on Mars has recently been emphasized by discoveries in Gale Crater by the Curiosity Rover (Grotzinger et al. Reference Grotzinger2014, Reference Grotzinger2015), which also include a sulphate-rich lacustrine mudstone unit. The mudstone contains traces of organic matter (Ming et al. Reference Ming2014) and a cross-cutting sediment injection structure (Grotzinger et al. Reference Grotzinger2014).
Lacustrine deposits on Earth are natural archives of life in and around lakes (Buatois & Mángano Reference Buatois and Mángano1998; Schnurrenberger et al. Reference Schnurrenberger, Russell and Kelts2003), preserved as abundant sedimentary organic matter and common carbonaceous fossils. Microfossils are commonly reported within lacustrine sediments including evaporitic sulphates (Schopf et al. Reference Schopf, Farmer, Foster, Kudryavtsev, Gallardo and Espinoza2012). This study sought to investigate whether lacustrine basins can additionally record the presence of the deep biosphere, i.e. microbial populations that develop within the sediments after burial, which might produce geochemical traces in several settings (Fig. 1). Bacterial sulphate reduction (BSR) is known to be a widespread metabolic process in the subsurface (McMahon & Parnell Reference McMahon and Parnell2014), where along with other microbial activity it is responsible for mineralogical alteration and neoformation (diagenesis) and is a common source of sulphide minerals (Cavalazzi et al. Reference Cavalazzi, Agangi, Barbieri, Franchi and Gasparotto2014). The iron sulphide pyrite occurs widely in shales in particular, where a supply of organic matter fuels microbial activity and biomass growth (Raiswell & Berner Reference Raiswell and Berner1986). In this study, sampling of sulphide minerals was undertaken in the Devonian Orcadian Basin, Scotland (Fig. 2), several aspects of which provide useful analogues for Mars. The basin was sulphate-rich, and it shows widespread evidence for sulphate precipitation at the sediment surface and sulphide precipitation in the subsurface (Muir & Ridgway Reference Muir and Ridgway1975; Duncan & Buxton Reference Duncan and Buxton1995). Because bacteria preferentially metabolize the lighter isotope of sulphur (32S), the sulphide minerals precipitated by BSR are characteristically depleted in the heavier isotope (34S; Machel Reference Machel2001). We therefore measured the sulphur isotope composition of the sulphides in order to assess if their precipitation was biologically mediated, and thereby indicative of deep biosphere activity, which would suggest that the possibility of deep biosphere activity also be considered in martian lacustrine basins.

Fig. 1. Schematic cross-section across a lacustrine basin, showing diversity of settings which may support subsurface microbial activity.

Fig. 2. Map of central part of Orcadian Basin, northern Scotland, showing localities for sulphide and sulphate samples. Outcrop of basement uplift occurs in SW Orkney. Localities A, Achanarras; B, Broadhaven; C, Castlehill; E, East Scapa; G, Graemsay; K, Ackergill; N, Breck Ness; P, Pennyland; S, Spittal; SH, Wick South Head; W, Warebeth; X, Staxigoe.
Geological setting
The Orcadian Basin is a 5+ km-thick package of continental sediments, deposited in an extensional basin of 10s of km wide during the mid-Devonian. The sediments include several kilometres thickness of lacustrine sediments, deposited in cycles reflecting fluctuations in water level and/or climate (Donovan Reference Donovan1980; Marshall & Hewett Reference Marshall, Hewett, Evans, Graham, Armour and Bathurst2003; Andrews & Trewin Reference Andrews and Trewin2010). The lake sediments are predominantly siltstones, which interdigitate with fluvial and aeolian sandstones towards the basin margins. A small proportion of the lake sediments is organic-rich and generated oil during burial (Trewin Reference Trewin1989; Duncan & Buxton Reference Duncan and Buxton1995). The sediments include widespread pseudomorphs after gypsum (Parnell & Janaway Reference Parnell and Janaway1990; Astin & Rogers Reference Astin and Rogers1991), indicating sulphate-rich pore waters. Offshore, sulphate evaporitic sediments are preserved unreplaced (Duncan & Buxton Reference Duncan and Buxton1995). In the middle of the basin is an inlier of the underlying crystalline basement (Strachan Reference Strachan2003), which is a palaeotopographic high comparable in nature to the central uplift found in larger impact craters (Kenkmann et al. Reference Kenkmann, Jahn, Scherler and Ivanov2005). As in craters (Osinski et al. Reference Osinski2013), the basement inlier was a focus of hydrothermal activity during sedimentation, including precipitation of base metal sulphide minerals (Gallagher et al. Reference Gallagher, Michie, Smith and Haynes1971; Plant et al. Reference Plant, Forrest, Hodgson, Smith, Stevenson, Thornton and Howarth1986). Further hydrothermal activity occurred after the cessation of sedimentation, evidenced by widespread calcite veins with traces of sulphides (Gallagher et al. Reference Gallagher, Michie, Smith and Haynes1971; McMahon et al. Reference McMahon, Parnell and Blamey2012; Dichiarente et al. Reference Dichiarente, Holdsworth, Dempsey, Selby, McCaffrey, Michie, Morgan and Bonniface2016). The lacustrine sediments, sulphate-rich chemistry and basement high are all characteristics pertinent to Mars. The rocks are well-exposed, and well-studied, and have proved valuable analogues for planetary science studies (McMahon et al. Reference McMahon, Parnell and Blamey2012; Hutchinson et al. Reference Hutchinson, Parnell, Edwards, Jehlicka, Marshall, Harris and Ingley2014).
Sulphide mineralization
The distribution of sulphide minerals in the Orcadian Basin indicates several settings where subsurface biological activity could have occurred, and which can be tested by isotopic analysis:
(i) Stratabound diagenetic. The earliest sulphides are pyrite nodules up to 10 cm size, which grew in the siltstones and sandstones during shallow burial but before significant compaction. They are locally abundant.
(ii) Sulphide mineralization that is directly associated with traces of degraded oil within porous sandstones. Oil was generated from the lacustrine sediments, probably during Carboniferous time and may have been degraded during subsequent Carboniferous-Permian uplift (Trewin Reference Trewin1989; Parnell et al. Reference Parnell, Carey and Monson1998). Syn-depositional hydrothermal. Hydrothermal activity which appears to have been syn-diagenetic, i.e. it involved rocks at and below the contemporaneous sediment surface. This includes the mineralization of buried stromatolites (cyanobacterial structures), by galena (lead sulphide) and sphalerite (zinc sulphide), indicating the influx of metals from outside the sedimentary environment, via a deep hydrothermal system. A well-documented example from Warebeth, SW Orkney shows sulphide replacement of the stromatolites before compaction occurred, i.e. very soon after sedimentation (Muir & Ridgway Reference Muir and Ridgway1975). The later stages of mineralization involve brittle fracture of lacustrine sediment around sulphide-bearing veins, indicating burial to the subsurface. Such deposits are focussed around the inlier of basement rock in SW Orkney, which may have channelled fluid flow and/or heat. Fluid inclusions in the sphalerite are monophase, indicating temperatures probably lower than 70°C (Goldstein & Reynolds Reference Goldstein and Reynolds1994), so within the tolerance of thermophile microbes. Syn-diagenetic sulphide precipitation similarly occurred in the northern part of the basin in the Shetland Islands at Matta Taing. At this site, centimetre-scale nodules rich in copper sulphides replaced organic-rich lake sediments, again before significant compaction (Hall & Donovan Reference Hall and Donovan1978).
(iii) Post-depositional hydrothermal. Later hydrothermal mineralization formed pyrite-bearing calcite veins (Fig. 3), which cut all rocks, indicating that they post-dated sedimentation in the basin. The veins occur particularly in the siltstones.
(iv) Sulphide mineralization associated with sediment fluidization and injection (Fig. 3a). As in other basins (Parnell et al. Reference Parnell, Boyce, Hurst, Davidheiser-Kroll and Ponicka2013), pyrite is found at the margins of injected sandstone structures that cut transgressively through the siltstones on a centimetre to metre scale.
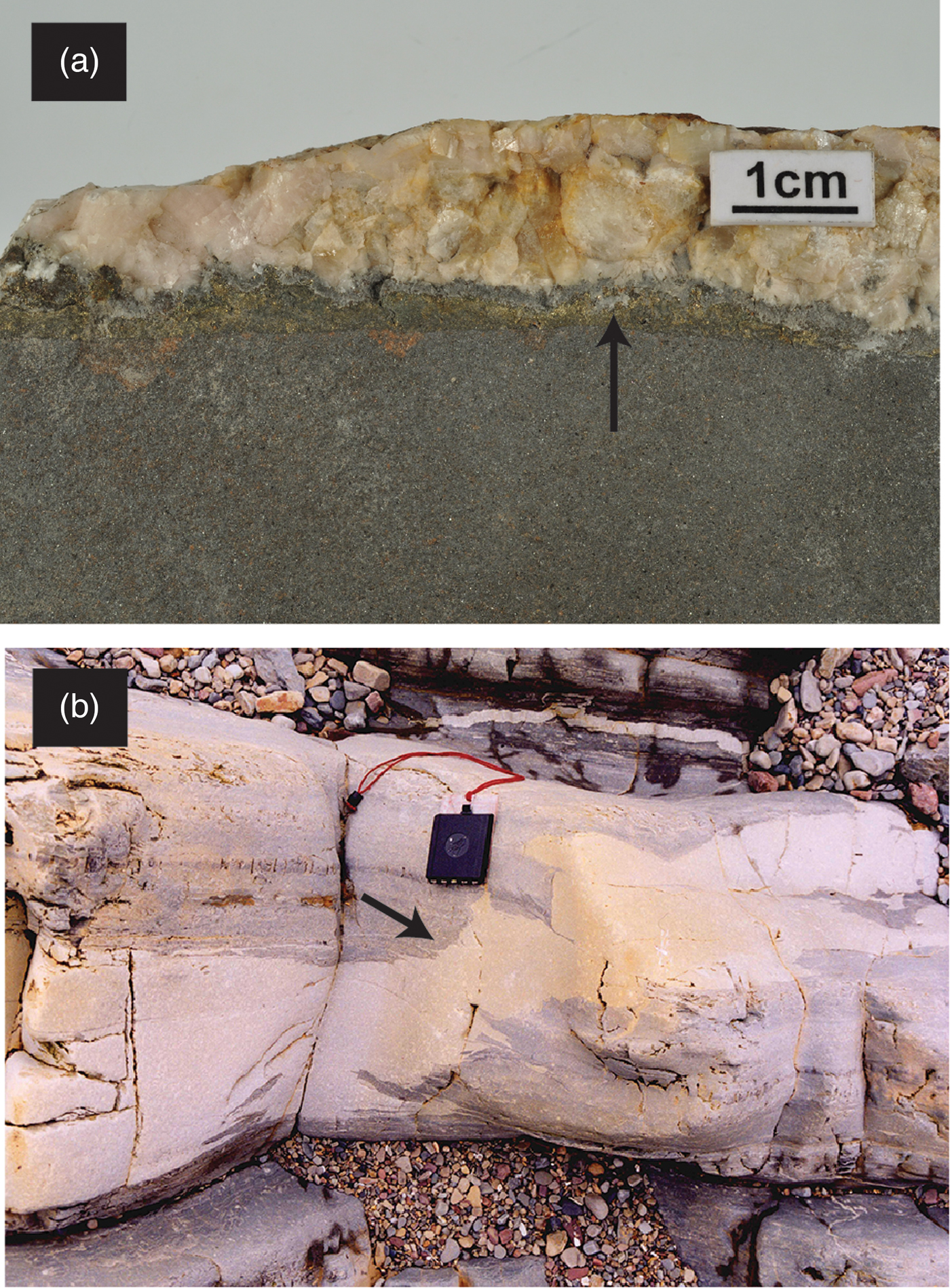
Fig. 3. Settings for sulphide mineralization in the sediments of the Orcadian Basin. (a) Margin of calcite vein through siltstone, with pyrite at interface (arrowed), Spittal. (b) Sandstone injection structure cross-cutting siltstone host, The Haven (transgressive relationship arrowed). Pyrite occurs at sandstone/siltstone boundary at a microscopic level. Compass scale 5 cm wide.
Methodology
Sulphide samples were prepared for conventional sulphur isotopic analysis by heavy liquid and hand picking techniques, at the Scottish Universities Environmental Research Centre (SUERC), East Kilbride. Heavy liquid separations were undertaken using suspension in bromoform. Sulphide separates were then analysed by standard techniques (Robinson & Kusakabe Reference Robinson and Kusakabe1975). Five to ten milligrams were utilized for isotopic analysis. SO2 gas was liberated by combusting the sulphides with excess Cu2O at 1075°C, in vacuo. Liberated gases were analysed on a VG Isotech SIRA II mass spectrometer, and standard corrections applied to raw δ66SO2 values to produce true δ34S. All SO2 gases were analysed on a VG Isotech SIRA II mass spectrometer. The standards employed were the international standard NBS-123 and IAEA-S-3, and SUERC standard CP-1. These gave δ34S values of +17.1, −31.6 and −4.6‰, respectively, with 1σ reproducibility better than ±0.2‰ around the time of these analyses. Data are reported in δ34S notation as per mil (‰) variations from the Vienna Canyon Diablo Troilite (V-CDT) standard.
Results
Sulphur isotope data
The isotopic composition of sulphur in 23 sulphide samples, together with a sample of gypsum from red bed sediments in the basin, is given in Table 1. The gypsum has a sulphur isotope composition (δ34S) of +19.5‰, which represents the sulphate in the depositional environment (i.e. the ‘parent’ for the sulphides). The sulphides have compositions in the range +18.5 to −26.7‰. The stratabound diagenetic samples (early diagenetic nodules in siltstones, pyrite in sandstones) have compositions similar to the parent sulphate (Fig. 4). Syn-depositional hydrothermal deposits from three localities, including replacive sulphides in shales and stromatolites, have compositions in the range +0.2 to −6.4‰. The post-depositional hydrothermal vein deposits have a wide range of composition from +13.8 to −26.7‰. No data were measured for the injectites.

Fig. 4. Histogram of sulphur isotope data from sulphides in the Orcadian Basin.
Table 1. Samples of sulphide and sulphate minerals used for sulphur isotope analysis

Discussion
Lacustrine basins and sediments
Sediments deposited in large lake basins have several characteristics which are favourable for microbial growth. They represent reservoirs for nutrients supplied by water flowing into the lake, incorporated into biomass and then released during decay below the sediment–water interface. Lacustrine sequences which consist of cyclic packages of sandstone and mudrock, such as in the Orcadian Basin (Donovan Reference Donovan1980; Andrews & Trewin Reference Andrews and Trewin2010), contain extensive interfaces between the sandstone and mudrock. The sandstone is porous and permeable enough to favour microbial colonization and transport, while the organic-rich mudrock would release nutrients during compaction, so the interface may be a favoured habitat for life (Parnell et al. Reference Parnell, Boyce, Hurst, Davidheiser-Kroll and Ponicka2013). As the sediments become compacted, their dewatering may cause deformation and mineralization of the fluid escape pathways, which could include biomineralization where springs emerge at the contemporary surface and precipitate their solutes. Such mineralized pathways would include fracture systems and sand injection structures. Many terrestrial lakes accumulate organic carbon, especially where the water column is seasonally or permanently stratified (Dean & Gorham Reference Dean and Gorham1998). All of these characteristics are feasible on early Mars, although the accumulation of organic carbon is likely to have been very limited.
Source of sulphides in Orcadian basin
There is widespread evidence for a sulphate-rich depositional environment in the Orcadian Basin, in pseudomorphs after gypsum that occur on many bedding planes (Parnell & Janaway Reference Parnell and Janaway1990; Astin & Rodgers Reference Astin and Rogers1991). This sulphate is an obvious source for the sulphides precipitated during burial, and a direct relationship can be observed where the original gypsum crystals are pseudomorphed by pyrite (Parnell & Janaway Reference Parnell and Janaway1990). Where sulphides formed before significant compaction, microbial sulphate reduction is strongly implicated, as the only widespread sulphide precipitation mechanism at low temperatures. Together with other examples of syn-diagenetic sulphides in algal breccias (Parnell & Janaway Reference Parnell and Janaway1990) and alkaline lake cherts (Parnell Reference Parnell1987), there is extensive evidence for the precipitation of sulphides in the shallow subsurface environment of the Orcadian lake basin. Thermochemical sulphate reduction, a non-biological mechanism, is effective at elevated temperatures from 80 to 100°C upwards, but does not generate strong negative isotopic fractionation (Machel et al. Reference Machel, Krouse and Sassen1995).
In the case of sulphides which formed after sedimentation was complete, i.e. those of post-Devonian age, sulphur could have been available from younger sources, in particular Carboniferous seawater. However, the post-Devonian history is more characterized by deep oxidative weathering (Robinson Reference Robinson1985). The close association of the late sulphide veins with the lacustrine siltstones rather implies that the sulphide and host carbonate were derived from within the siltstone rather than by downwards percolation through a thick pile of sediments.
The ‘stratabound’ group of sulphide samples yield data very similar to the parent sulphate. The similarity is not diagnostic of a specific mechanism of sulphate reduction. It is likely that these sulphides were a product of microbial sulphate reduction, given the low temperatures typical of early diagenesis, but this is not conclusively proven by the isotope data. The vein sulphide samples, by contrast, exhibit a fractionation to a broad range of lighter (more negative) compositions. The lightest sample, at −26.7‰ has a fractionation of −45‰ from the parent sulphate. The broad range and the maximum fractionation are both characteristic features of microbial sulphate reduction (Machel Reference Machel2001). The syn-depositional hydrothermal samples have a smaller range of compositions than the post-depositional hydrothermal samples. They all have a fractionation of about 20‰ from the parent sulphate, which is typical for microbial sulphate reduction in an open system. The greater variation in deep hydrothermal samples represents a greater variation in fluid compositions, and probably variation in the timing of sulphide precipitation. It is likely that the stratabound sulphides were also a product of microbial sulphate reduction, given the low temperatures typical of early diagenesis, but that is not conclusively proven by the isotope data.
Analogue for Mars
Lake sediments may be widespread on Mars (Cabrol & Grin Reference Cabrol and Grin2010), including in the Valles Marineris canyon region (Lucchitta Reference Lucchitta, Cabrol and Grin2010; Warner et al. Reference Warner, Sowe, Gupta, Dumke and Goddard2013) and the northern plains (Parker et al. Reference Parker, Grant, Franklin, Cabrol and Grin2010), and particularly in craters. The lacustrine sediments of the Orcadian Basin are a valuable analogue for those on Mars. Gale Crater, at 140 km width, is of comparable size to the Orcadian Basin (Trewin Reference Trewin1989). The lake system in Gale Crater lasted from thousands to millions of years (Grotzinger et al. Reference Grotzinger2015), also comparable with the long history of Orcadian lacustrine sedimentation (Andrews & Trewin Reference Andrews and Trewin2010; Andrews et al. Reference Andrews, Cornwell, Trewin, Hartley and Archer2016).
Sulphate minerals are widely distributed on Mars (Ehlmann & Edwards Reference Ehlmann and Edwards2014), so the sulphate-rich nature of the lake waters and sediments of the Orcadian Basin make the deposits an especially pertinent analogue. The occurrence of some sulphides in degraded oil highlights a limitation to the analogy with Mars or other planets. In this case, microbial activity has used hydrocarbons as a source of carbon and energy (as is widely observed in oil reservoirs), and the hydrocarbons exist only as a consequence of earlier carbon fixation by photosynthesis in surface waters. Traces of hydrocarbons occur in the sulphide-bearing veins, so could have supplied carbon to microbial life. Nevertheless, the mineral veins in the Orcadian Basin demonstrate that hydrothermal systems can develop in a lacustrine basin, and circulate fluid and nutrients both during sedimentation and subsequent to it. The post-sedimentation hydrothermal activity is not mere happenstance; it is a natural consequence of the expulsion of fluid from a thick, geothermally heated pile of compacting sediment in a long-lived basin. If there is any microbial activity, hydrothermal settings would be preferred habitats as they focus nutrient availability and recharge, and activity is promoted at elevated temperatures. This much can be translated to the exploration of Mars, where the case for investigating the sediment fills of crater basins has been well made. Although crater lakes would not undergo continued subsidence like the extensional Orcadian Basin, the thermal afterglow of the impact itself is expected to have driven hydrothermal activity in craters on Mars as it has on Earth (Rathbun & Squyres Reference Rathbun and Squyres2002). This is thought to explain the occurrence of post-impact silica and sulphate veins observed by rovers in Endeavour Crater and Gale Crater on Mars (Arvidson et al. Reference Arvidson2014; Grotzinger et al. Reference Grotzinger2014). The focus of hydrothermal activity around a basement inlier in the Orcadian Basin is especially pertinent, as this feature is found in many impact craters (e.g. Mount Sharp in Gale Crater) and has been identified as a desirable target for astrobiological sampling on Mars.
The Orcadian injected sandstone structures (Fig. 3b) are centimetre-scale width and transgress the stratigraphy, similar to the injected structure in Gale Crater as shown by Grotzinger et al. (Reference Grotzinger2014). Lacustrine successions commonly contain alternating sandstone and mudstone beds, and disturbances (such as meteorite impacts) could trigger gravitational instability stimulating injections of the sandstones through the mudstones. Injected structures are conduits for fluid flow, and have a high surface area of sandstone/mudstone interface, making them good habitats (Parnell et al. Reference Osinski2013). Such interfaces also occur from where sand fills crack-like structures in adjacent mud layers, which can open spontaneously during burial and are observed (lithified) both in the Orcadian Basin and in Gale Crater (Donovan & Foster Reference Donovan and Foster1972; Grotzinger et al. Reference Grotzinger2014; McMahon et al. Reference McMahon, van Smeerdijk Hood and McIlroy2016). Injection and crack-like structures therefore deserve close attention in the search for life.
Both hydrothermal veins and injection structures penetrate to depths of 1–2 km in sedimentary basins, a depth range that includes most deep biosphere activity (McMahon & Parnell Reference McMahon and Parnell2014). On Mars, where gravitational compaction and geothermal gradients are milder than on Earth, there is no reason to believe that a deep biosphere could not extend to these depths where liquid water is available (Michalski et al. Reference Michalski, Cuadros, Niles, Parnell, Rogers and Wright2013).
The application of sulphur isotopes in this study has served to prove BSR at depth in the Orcadian Basin, and this is the possibility of deep life in lacustrine basins on Mars. The remote measurement of sulphur isotopes on samples on Mars could also have value in the exploration for biological activity there (Franz et al. Reference Franz, Mahaffy, Kasprzak, Lyness and Raaen2011). However, the fractionation of sulphur isotopes 32 and 34 that is the basis of our conclusion of BSR has evolved over billions of years and is not normally evident in Archean samples on Earth. Evidence of biological activity is nonetheless possible in Archean samples using rarer sulphur isotopes 33 and 36 (Halevy Reference Halevy2013; Marin-Carbonne et al. Reference Marin-Carbonne, Rollion-Bard, Bekker, Rouxel, Agangi, Cavalazzi, Wohlgemuth-Ueberwasser, Hofmann and McKeegan2014). The potential of interrogating Archean sulphide samples is especially pertinent given the equivalent Archean age for the lacustrine samples on Mars. As an understanding of the sulphur isotope systematics on Mars improves, so will the evaluation of whether any anomalous isotopic fractionation could be used as an indicator of possible biology.
Conclusions
The lacustrine sediments of the Orcadian Basin exhibit evidence for a deep biosphere in several contexts, including syn-depositional hydrothermal deposits, post-depositional hydrothermal deposits and injected sandstones. Hydrothermal deposits are particularly focussed around a basement uplift. Each of these contexts has potential relevance to Mars. The evidence for microbial activity in these settings in the Orcadian Basin is in sulphide precipitation, some of which has diagnostic sulphur isotope compositions. Although the surface of Mars is sulphate-rich, and sulphide precipitation would be a feasible signature of microbial activity there, this is not essential to the analogy. The critical conclusion is that a lacustrine basin contains deep biosphere habitats, and the same habitats could occur on Mars.
Acknowledgements
The sample of gypsum was kindly provided by John Marshall, University of Southampton. SM was funded by an STFC Aurora studentship (grant ST/1506102/1) and by the NASA Astrobiology Institute (NNA13AA90A Foundations of Complex Life). AJB is funded by NERC support of the Isotope Community Support Facility at SUERC.