Milk is a valuable source of human nutrition. Prior to consumption and due to safety requirements and consumer preferences, the milk is normally treated by pasteurisation, homogenisation, spray drying and/or other processes (Köhlera and Schuchmann, Reference Köhlera and Schuchmann2011; Li et al., Reference Li, Ye and Singh2021). Homogenisation helps stabilise the cream to produce a uniform solution and can change the taste and mouthfeel properties of the drink (Köhlera and Schuchmann, Reference Köhlera and Schuchmann2011). Heat treatments have been shown to affect nutritional and digestion characteristics of milk (Wada and Lönnerdal, Reference Wada and Lönnerdal2014). Clot formation in the stomach is affected by heat and pressure especially at temperatures that denature whey proteins. The denaturated proteins join the casein proteins to produce a softer clot that proceeds through gastric digestion quicker (Li et al., Reference Li, Ye and Singh2021).
During pasteurisation and homogenisation, different components of milk interact with each other and cause changes in micelle and protein structure. Although the whey fraction only makes up approximately 20% of the total protein content, this fraction is of great nutritional importance because of the high levels of two essential amino acids, tryptophan and lysine (Elliott and Elliott, Reference Elliott and Elliott2001; Michalski and Januel, Reference Michalski and Januel2006; Li et al., Reference Li, Ye and Singh2021) and is prone to protein modifications due to the mentioned pre-consumption treatments, which may affect how the proteins are digested and absorbed in the body (van Lieshout et al., Reference van Lieshout, Lambers, Bragt and Hettinga2020). Whey proteins have denaturation temperatures less than 100°C whereas caseins remain stable until over 100°C (Michalski and Januel, Reference Michalski and Januel2006). The denaturation of the whey proteins during treatment allows for more exposed areas of the protein that can then be chemically modified.
Raw milk contains casein micelles and milk fat globules. The caseins bind together to form micelles through several different chemical bonds including hydrogen, ionic, disulphide and hydrophobic interactions. Proteins in casein micelles are structured so that the hydrophobic proteins, α- and β-caseins, are within the micelle and the κ-casein hydrophilic tail is positioned on the outer part of the micelle. The presence of salts, specifically Ca2+, form ionic bonds with κ-casein tails to stabilise the casein micelles and keep them in solution (Roach and Harte, Reference Roach and Harte2008). They also contain minerals including calcium, magnesium, phosphorus and citrate (Michalski and Januel, Reference Michalski and Januel2006). The milk fat globule consists of triacylglycerols in the centre surrounded initially with xanthine oxidase protein and phospholipids followed by a bilayer membrane. This bilayer membrane contains glycosylated polypeptides, phospholipids, cholesterol and butyrophilin protein. Milk fat globule membranes in raw milk are an average of 4 μm but can be as large as 20 μm in diameter (Michalski and Januel, Reference Michalski and Januel2006).
Previous studies have shown that the total protein in the cream fraction increases with pasteurisation and homogenisation (Zamora et al., Reference Zamora, Ferragut, Guamis and Trujillo2012; Kiełczewska et al., Reference Kiełczewska, Ambroziak, Krzykowska and Aljewicz2021). During homogenisation, milk fat globules break up into smaller fat globules and have been shown to reduce to a similar size as the casein micelles when homogenised at 80–200 bar (8 MPa–20 MPa) (Lee and Sherbon, Reference Lee and Sherbon2002; Michalski and Januel, Reference Michalski and Januel2006; Zamora et al., Reference Zamora, Ferragut, Guamis and Trujillo2012; Ren et al., Reference Ren, Li, Liu and Ma2020). During heat treatment, milk forms casein–whey complexes and with homogenisation, β-lactoglobulin and casein proteins integrate into the milk fat globule membrane (MFGM) to form fat–protein complexes (Michalski and Januel, Reference Michalski and Januel2006). Proteomics involves examining a comprehensive selection of proteins (such as proteins from milk) and generally involves mass spectrometry for identification.
Pressure and/or heating of milk may cause protein modifications which can affect the taste and nutritional value of milk (Korhonen et al., Reference Korhonen, Pihlanto-Leppäla, Rantamäki and Tupasela1998; Calvano et al., Reference Calvano, Monopoli, Loizzo, Faccia and Zambonin2012). Although the Maillard pathway has been studied extensively, other types of modifications have been studied less (Li et al., Reference Li, Ye and Singh2021). This study's aims were to examine how heat and pressure, or pasteurisation and homogenisation individually influence protein modifications as well as to determine the extent of consistency in the location of the modification.
Materials and methods
Milk treatment
Fresh whole bovine milk was collected and prepared as stated previously (Reis et al., Reference Reis, Harris, Berry, Nguyen, Maclean and Weeks2020). Briefly, raw milk was pasteurised at 72°C for 15 s using two coiled stainless-steel tubes connected in series and immersed in hot water baths. Homogenisation was performed using a 1-stage homogeniser (Avestin Emulsiflex C5). To avoid batch variation as observed in previous studies (Rodríguez-Alcalá et al., Reference Rodríguez-Alcalá, Castro-Gómez, Felipe, Noriega and Fontecha2015) the same bulk milk sample was processed under the 4 processing conditions, heat and heat plus pressure investigated in this study: (1) no pressure at 45°C; (2) 35 MPa at 45°C; (3) no pressure at 80°C; (4) 35 MPa at 80°C, where no pressure refers to no additional pressure applied. Samples 1 and 3 were run with no additional pressure so effects of the homogenisation pressure could be determined separately from the homogenisation temperature. Each homogenisation treatment condition was done with 3 passes through the homogeniser. Samples of the raw and pasteurised milk were also kept for analysis. The milk samples were cooled and stored at 4°C overnight. Milk collection and processing occurred on the same day within 12 h. Additionally, to avoid experimental bias, processing conditions were randomised.
Microstructural analysis
The microstructural analysis of milk samples was carried out using an inverted confocal laser scanning microscope (CLSM) (Fluoview FV10i, Olympus, Auckland, New Zealand). Milk was mixed with Fast Green FCF (1 mg/ml) and Nile Red (1 mg/ml) and stained for at least 1 h at room temperature. An aliquot of 5 ml stained milk was then mixed with 20 ml of a low melting point agarose solution before deposition onto a cavity microscope slide and covered with a 0.17 mm thick coverslip (ProSciTech, QLD, Australia). The × 60 objective and numerical aperture of 1.0 were used. The excitation/emission wavelengths were set at 480 nm/ 500–530 mm and 635 nm/660–710 nm for Nile Red and Fast Green FCF, respectively. At least six images were taken for each milk sample and the typical images are presented in the results section. More detailed information can be found in the online Supplementary File methods and materials.
Sample preparation for proteomics
Cream was separated from the homogenised milk using a sugar gradient according to a method based on that of Lee & Sherbon (Lee and Sherbon, Reference Lee and Sherbon2002). Cream was collected and dried at room temperature. The skimmed milk was separated into casein and whey using ultracentrifugation as published previously (Gathercole et al., Reference Gathercole, Reis, Agnew, Reis, Humphrey, Harris, Clerens, Haigh and Dyer2017) by centrifuging at 100 000g for 1 h to limit the changes that occur due to acid precipitation. During acidification, the acid reduces the amount of calcium bonding on κ-casein which leads to changes in the casein micelle structure (Li and Zhao, Reference Li and Zhao2019).
Proteins were denatured in a urea buffer (7 M urea, 2 M thiourea and 50 mM dithiothreitol) with shaking overnight at 25°C. To isolate the proteins, methanol–chloroform extraction was done according to the method by Wessel and Flügge (Wessel and Flügge, Reference Wessel and Flügge1984). The precipitated proteins were left to air dry.
The protein precipitate was dissolved in 60 μl of 0.1 M ammonium bicarbonate. Proteins were reduced with 100 mM tris(2-carboxyethyl)phosphine for 45 min at 56°C on a thermomixer. The proteins were then alkylated with 150 mM iodoacetamide in 50 mM ammonium bicarbonate and incubated in the dark at room temperature for 30 min with shaking. Proteins were digested with trypsin (Promega) with a ratio of 1 μg trypsin: 50 μg of protein, overnight at 37°C with shaking.
The digests were dried and resuspended in 100 μl of 10 mM ammonium formate, pH 10. To clean the sample with Empore C18 material, the disks were conditioned for 1 min each with acetonitrile followed by methanol and then water. Three disks were then placed directly into each sample and incubated to bind the peptides to Empore disks for 2.5 h at room temperature with vortexing. Prior to eluting the Empore disks were rinsed in 0.1% formic acid. The peptides were eluted in two fractions. Initially the disks were placed in 100 μl of 10 mM ammonium formate in 10% v/v acetonitrile and vortexed for one hour. The disks were then placed in 100 μl of 10 mM ammonium formate in 50% v/v acetonitrile for one hour. The disks were discarded, and each eluent was dried using a centrifugal concentrator and stored at −20°C until LC-MS/MS analysis. More detailed information can be found in the online Supplementary File methods and materials under sample preparation for proteomics.
LC-MS/MS analysis
Samples were separated in 0.1% formic acid on a Bruker Nanoadvance LC with a ProntoSIL C18AQ 100 μ × 150 mm column with 3.0 μm particles and 200 Å pore size (nanoLCMS Solutions). The column was connected via captive spray ioniser (Bruker Daltonics) to an Amazon ETD ion trap MS (Bruker Daltonics). A multistep gradient was used to separate the sample between 2 and 45% mobile phase B. Mobile phase A was 0.1% formic acid in LC-MS grade water and mobile phase B was 0.1% formic acid in LC-MS grade acetonitrile. For the milk fat globule (cream), a slightly different gradient was used for separation (see supplementary material for further details including information on both gradients). In all runs, MS was run in positive mode between 350–1200 m/z with auto MS/MS on up to 10 precursors. Active exclusion of ions was in place after 1 spectra for 0.2 min unless intensity was increased at least five fold.
Protein and peptide identification
The two Empore fraction spectra were combined and searched in ProteinScape (Version 4.0.3 315, Bruker Daltonics). Spectra were compared against the SwissProt Bos Taurus database using Mascot and ProteinExtractor and six different sets of modifications (for details see the protein and peptide identification section of the online Supplementary File). For all searches, semitrypsin was selected as the enzyme allowing for up to 2 missed cleavages. The peptide tolerance was 0.1 Da and the MS/MS tolerance was 0.6 Da was used. All searches contained fixed carbamidomethyl of Cys and variable deamidation (Asn or Gln) and phosphorylation off Ser or Thr. Proteins observed only in the homogenised milk cream fraction were analysed with Panther (Thomas et al., Reference Thomas, Kejariwal, Guo, Mi, Campbell, Muruganujan and Lazareva-Ulitsky2006; Mi et al., Reference Mi, Muruganujan, Ebert, Huang and Thomas2018) to determine molecular and biological functions.
Protein modification analysis
Modification scores, to determine the degree of protein modifications, were determined using an in-house software as reported previously (Dyer et al., Reference Dyer, Plowman, Krsinic, Deb-Choudhury, Koehn, Millington and Clerens2010; Lassé et al., Reference Lassé, Deb-Choudhury, Haines, Larsen, Gerrard and Dyer2015; Gathercole et al., Reference Gathercole, Reis, Agnew, Reis, Humphrey, Harris, Clerens, Haigh and Dyer2017). Further information on this process can be seen in the protein modifications analysis section in the online Supplementary File. Modification scores were calculated for each type and group of modifications (e.g. carboxymethylation, oxidation of cysteine and total oxidation) as well as a total modification score. The average of the three replicates was used in further analysis. The Sparkline function in Microsoft Excel was used to screen for modifications which differed between milk treatments. The most abundant types of modifications were investigated further to determine if the modification site or area of the protein was consistent. One-way ANOVA was done on total cysteine oxidation, total proline oxidation and total oxidation for all three fractions. If the modification score means for treatments were significantly different according to ANOVA, pairwise comparisons were run using the Holm–Skdak method (SigmaPlot version 13.0, Dundas Software ltd, Germany) to determine significant differences between treatments. Significance was considered as P < 0.05 or better.
Results and discussion
Microstructure analysis of milk samples
Confocal microstructural analysis was done to examine processing differences in the milk fat globule and the locality of proteins and fats in the samples. A stronger processing effect was observed with the milk samples treated with heat and pressure compared to those that were only heat treated (Fig. 1). As expected, homogenisation led to a decrease in the fat globule size and absorption of proteins onto the fat globule surface (Fig. 1d, 1f). This observation was supported by the fact that the number of proteins identified in the homogenised cream fractions was up to 3 times higher than the number found in the raw sample (see Table 1). The pasteurised cream fraction (without any further pressure or heat treatment) contained the least average number of proteins (119) in the processed samples. These results suggest that the majority of protein additions into the cream fraction occurred during homogenisation by being absorbed onto the milk fat globule surface, where the native membrane was lost. This has also been observed previously (Kiełczewska et al., Reference Kiełczewska, Ambroziak, Krzykowska and Aljewicz2021). The highest number of proteins were identified in the sample homogenised at 35 MPa and 45°C with an average of 325 proteins identified (see Table 1).

Fig. 1. Microstructure of milk samples treated at different temperatures and homogenisation pressures as observed by CLSM. (a) Raw; (b) pasteurised (72°C, 15 s); (c) 45°C, no applied pressure; (d) 45°C, 35 MPa; (e) 80°C, no applied pressure; (f) 80°C; 35 MPa. Nile Red stained fat appears red; FCF stained protein appears green. Arrow indicates whey protein aggregates. CLSM images were captured using a 60 × objetive and a 4 × digital zoom. The scale bars are 5 mm in length.
Table 1. Average number of protein identifications in cream after pasteurisation, homogenisation and pressure treatments
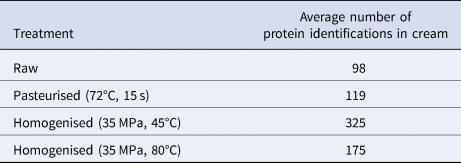
Homogenisation at 35 MPa and 80°C resulted in smaller fat globules than homogenisation at 35 MPa and 45°C (Fig. 1f v. 1d), suggesting that higher temperature during homogenisation could increase the efficiency of homogenisation. Subtle differences in the microstructure were observed among milk samples treated at different temperatures including raw, pasteurised (72°C, 15 s) and samples processed at different temperatures but without any pressure (45°C v. 80°C) (Fig. 1a–e). Some protein aggregates, however, were observed in the sample at 80°C (Fig. 1e) due to the denaturation of whey proteins at this temperature (Wijayanti et al., Reference Wijayanti, Bansal and Deeth2014). Previous work has shown that denaturation of whey proteins increased from 28.34 to 45.37% when treatment temperature increases from 65 to 85°C (Qian et al., Reference Qian, Sun, Cao, Tuo, Jiang and Mu2017). Fewer proteins were found in the cream fraction of the high temperature (80°C) homogenised samples compared to the lower temperature (45°C). This was likely due to the occurrence of protein aggregates at 80°C (Fig. 1e), which led to insufficient denaturation during the urea step and thus reduced trypsin digestion and thus less protein identifications when the milk homogenised at 80°C was compared to 45°C. Urea denaturing conditions during sample preparation were not done at 25°C in this work to limit further protein modifications.
General modification trends
When milk is processed, a number of protein modifications occur, including Maillard products and oxidation of different amino acid sites in proteins (Dyer et al., Reference Dyer, Clerens, Grosvenor, Thomas, Callaghan, Deb-Choudhury and Haines2016; Gathercole et al., Reference Gathercole, Reis, Agnew, Reis, Humphrey, Harris, Clerens, Haigh and Dyer2017). We were unable to separate the cream from the milk homogenised at 80°C and 35 MPa so the milk fat fraction from this treatment was not analysed for proteins and protein modifications. The inability to separate the cream suggests increase of pressure and temperature changes the milk fat globules to the extent they cannot be separated even with the density gradient used. Variations in the degree of protein modifications were observed across the treatments but the response differed between fractions (whey, casein and cream) (See Fig. 2 for comparisons of total cysteine oxidation, total proline oxidation and total oxidation). Generally, modifications increased in samples with heat treatment compared to the raw, particularly more evident for the cream and whey fractions, which suggests that the proteins in these fractions had more exposed amino acids for modification reactions to occur. Since heat denatures whey proteins, the increase in amino acid exposure could be due to the unfolding of the proteins. For some modifications, there was a trend in the cream fraction that applying pressure (35 MPa) led to lower modification scores than when heated to the same temperature with no applied pressure. Proline oxidation showed a trend of increasing when homogenisation occurred at 45°C and 35 MPa but reducing when it was at 80°C and 35 Mpa, both compared to just heat treatment at 80°C (Fig. 2d–2f).
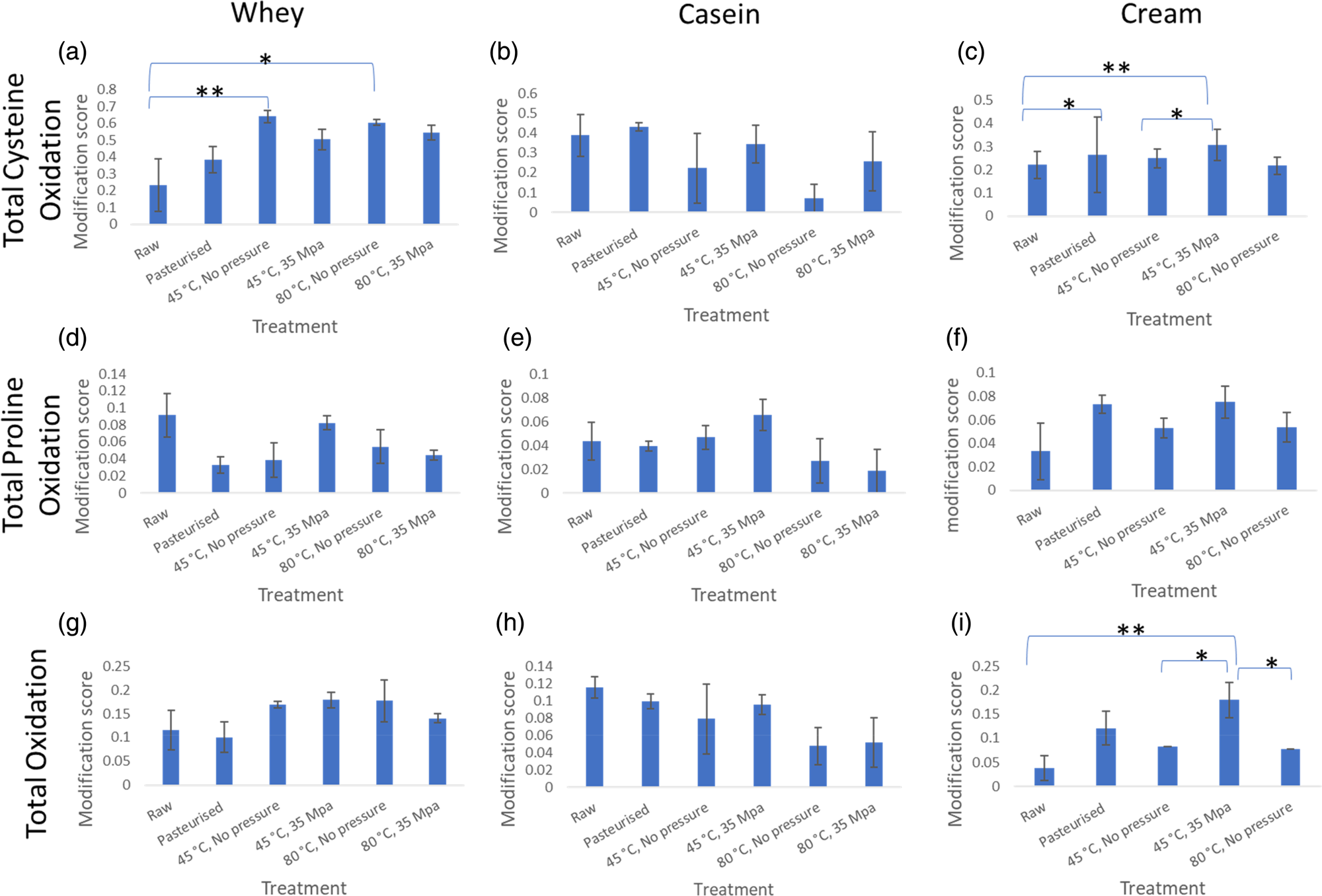
Fig. 2. Average protein modification score for total cysteine oxidation, total proline oxidation and total oxidation modifications. This represents the degree of the presence of these modifications after the different treatments. Error bars are represented by ± standard error. (a) refers to total cysteine oxidation in whey, (b) is total cysteine oxidation in casein, (c) is total cysteine oxidation in cream, (d) refers to total proline oxidation in whey, (e) is total proline oxidation in casein, (f) is total proline oxidation in cream, (g) refers to total oxidation in whey, H is total oxidation in casein, I is total oxidation in cream. * refers to 0.05<P < 0.1 and ** refers to P < 0.05 according to one-way ANOVA.
Numerical differences in cysteine oxidation were determined in whey and cream fractions and for total oxidation in cream, but one-way ANOVA showed these to be non-significant (0.05 < P < 0.1). The heated samples had a significantly higher amount of cysteine oxidation, compared to the raw, in the whey fraction (Fig. 2a). In the cream fraction, there was a significantly (P < 0.05) higher amount of cysteine modification in the homogenised sample (45°C, 35 MPa) compared to raw. Numerical differences were also observed between raw and pasteurised and heated at 45°C, no applied pressure as well as raw and the 45°C, 35 MPa treatments.
Looking at the total oxidation of proteins in the cream (Fig. 2i), there was a significant difference between the total oxidation modifications between raw and homogenised (45°C, 35 MPa) samples which may be explained by the addition of proteins that are prone to oxidation. These protein changes to the MFGM occur during homogenisation which involves temperature and pressure treatment. Cream fractions from the samples heated without pressure were numerically different to the total oxidation found in the homogenised sample (sample with pressure applied, 45°C, 35 MPa) showing that both the heat and the pressure affect protein modifications in milk.
The decrease in the number of proteins identified in the cream fraction of sample 80°C, 35 MPa (Table 1), could be explained by the protein assembly as they may have been too aggregated to pull apart in the urea denaturation buffer and allow access for efficient trypsin digestion. Further work to fully understand this is required but was out of scope for this project.
Michalski and Januel have questioned whether homogenisation might be beneficial health-wise (Michalski and Januel, Reference Michalski and Januel2006). Since then a human ex vivo study showed that homogenisation does have a beneficial effect as it increases the digestion of proteins and lipids (Islam et al., Reference Islam, Devle, Comi, Ulleberg, Rukke, Vegarud and Ekeberg2017). Our results showed some benefits in homogenisation protecting the milk from treatment related modifications which is illustrated in the online Supplementary Fig. S1 for α-S1-casein and β-lactoglobulin for the extreme homogenisation treatment (80°C, 35 MPa). Results from the confocal microscopy showed that the fat globules decreased in size and there was increased coating of proteins onto the globule. This could reduce access to the proteins to oxidative conditions and other chemicals required for protein modifications.
Milk fat globule membrane protein changes
To determine if the number of proteins in the cream fraction changes due to processing, we compared the number of proteins identified in the cream fraction for raw, pasteurised, 45°C, no applied pressure and 45°C 35 MPa treatments, with a Venn diagram (Venny 2.1.0, http://bioinfogp.cnb.csic.es/tools/venny/: Fig. 3). A total of 194 (23.9%) of the proteins were found in all treatments. These proteins included the caseins (α-S1-casein, α-S2-casein, β-casein and Κ-casein), lactadherin (only found in whey and casein when treated at 80°C) and xanthine dehydrogenase/oxidase (XDH), an MFGM structural protein. Previous work has shown that an increase in casein and whey proteins are found in milk fat droplets after milk is pasteurised and homogenised which explains the increase of protein identifications seen in Table 1. Heat has been shown to increase the disulphide bonds between MFGM proteins and other proteins. Further to this, it has been suggested that the increased surface area caused by the reduced milk fat globule size after homogenisation is obtained through immersion of whey and casein proteins (Zamora et al., Reference Zamora, Ferragut, Guamis and Trujillo2012).

Fig. 3. Venn diagram of cream proteins found in all three replicates for each treatment.
There were 91 proteins that were observed in all homogenised samples (45°C, 35 MPa) but were not always observed in the other treatment groups (See online Supplementary File). These proteins included latrophilin-1, vasculin, mucin-15 and two calcium channel proteins: trimeric intracellular cation channel type B and calcium/calmodulin-dependent protein kinase type II β. Latrophilin is involved in molecular binding between proteins (Meza-Aguilar Diana and Boucard Antony, Reference Meza-Aguilar Diana and Boucard Antony2014) and may help in the formation of the homogenised MFGM. Vasculin is a transcription factor according to UniProt (Consortium, Reference Consortium2018) but its presence in milk has not been discussed in the literature as far as we are aware. Mucin-15 is a membrane bound glycosylated protein and has previously been found in raw milk (0.80 μg/mg) and in raw MFGM (15 μg/mg) (Pallesen et al., Reference Pallesen, Pedersen, Petersen and Rasmussen2007) although in this current study it was only found in raw and heated casein fractions and the whey samples from milk homogenised at 80°C and 35 MPa. Research in the past has shown that calcium is present in the casein and whey fractions rather than cream but this research has been done predominantly on raw milk (Neville et al., Reference Neville, Keller and Casey1994). In homogenised cream though, the natural milk fat globule is changed (Hillbrick et al., Reference Hillbrick, Augustin and Udabage2006). The presence of calcium channel proteins appearing in the membrane post homogenisation suggests that the presence of calcium channels allows Ca2+ ions into the MFGM.
The function of the proteins was searched for using Panther. A number of proteins were associated with DNA folding including histone H1.3 and H1.2, hepatocyte nuclear factor 3-γ and heat shock protein HSP 90-beta (HS90B). These binding proteins may help with the integration of more proteins into the milk fat globule although further work is required to substantiate this.
Protein coverage and modification changes
A selection of proteins was examined to see the distribution of coverage for each treatment and the location of oxidation and Maillard modifications (see online Supplementary File). When combining the coverage from all three fractions (cream, casein and whey) the same parts of the protein were observed in the proteomics results for all treatments. For example, in α-S1-casein, amino acids 1–16, 74–94 and 167–190 were not observed in any of the samples. Amino acids 1–15 form the signal peptide and are normally removed prior to secretion. Detection of this section of the sequence is unexpected, therefore (Consortium, Reference Consortium2018). Trypsin cleavage of Arg16 could explain the absence of that peptide in the results since only peptides with ≥5 amino acids were identified using these proteomic methods. For α-S1-casein, the highest sequence coverage was observed in the casein fraction. Maillard modifications were most dominant on Lys117, 118, 120 for the casein and cream fractions. Phe38 and 39 were dioxidised in all samples, except for the those prepared at 80°C (without pressure or pressurised at 35 MPa) in the casein fraction, which suggests that phenylalanine may have been further oxidised to DOPA-derived quinone (F + 2O–2H) or DOPA-derived hydroxyquinone (F + 3O–2H).
For β-lactoglobulin the whole protein sequence minus the signal peptide (amino acids 1–16) (Consortium, Reference Consortium2018) was observed in at least one of the processed milk treatments. Maillard modifications were consistently observed on the lysines between amino acid location 85 and 99. According to UniProt, this is an area of beta sheet and is located on the outside of the tertiary structure (Consortium, Reference Consortium2018) which explains why these lysines are prone to modification. These Maillard modifications were found in the casein and whey fractions but not in the cream fraction. β-lactoglobulin has previously been shown to integrate into the MFGM due to homogenisation but we observed it also in the cream fraction in all samples analysed including raw. The largest sequence coverage of β-lactoglobulin in the cream fraction was observed in the sample heated to 80°C without additional pressure. This is in agreement with previous studies that show that not only pressure but other factors such as high temperature also lead to the integration of this protein into the cream fraction, as part of the MFGM. Denatured β-lactoglobulin due to heat treatment has been reported to be able to bind to the MFGM (Lee and Sherbon, Reference Lee and Sherbon2002).
Two cream proteins, XDH and lactadherin, showed fewer modifications than the more dominant milk proteins α-S1-casein and β-lactoglobulin. XDH is a large protein of 1332 amino acids but showed limited modifications. This protein was found to be dioxidised at Trp236 except in the homogenised sample and other protein modifications were limited. XDH was only identified in the cream fraction. Lactadherin was only found in the cream fraction until heated to 80°C where one peptide, ETQYVRLVPI ICHR, was found in whey when no pressure was applied with the heating, and two peptides, EYLKTFK and VTGIITQGAR were found in the casein fraction when heated to 80°C under 35 MPa pressure. These results suggest that lactadherin and XDH remain an integral part of the milk fat globule even after homogenisation.
The reduced modifications in XDH compared to the whey and casein proteins can be explained by its position within the milk fat globule. The XDH protein is present between the double polar lipid membrane on the outside of the globule and the cholesterol layer that surrounds the triglyceride core (Affolter et al., Reference Affolter, Grass, Vanrobaeys, Casado and Kussmann2010). This position would protect the protein from modifications. Lactadherin had a small number of oxidations throughout the protein. Lactadherin (also known as PAS 6/7) is implanted in the outside of the double polar lipid membrane with a tail protruding outwards from the milk fat globule along with other biomolecules (Evers, Reference Evers2004; Affolter et al., Reference Affolter, Grass, Vanrobaeys, Casado and Kussmann2010). Its limited protein modifications suggest that even through it protrudes outwards it is still protected from protein modifications, possibly by the other biomolecules protruding from the milk fat globule.
In conclusion, treatment of milk prior to consumption causes protein modifications that could affect the nutritional value of milk. This work found that both heat and pressure applied in homogenisation resulted in significant protein modifications in milk, which differed according to the milk fractions, whey, casein and cream. We found that extreme homogenisation pressure and temperature (35 MPa, 80°C) changes the structure of cream to the point that we could not separate it from the milk. Temperature also has an effect in the extent of protein modification suggesting that homogenising at low temperatures will reduce protein modifications. In addition, we observed that modifications often occurred to the same amino acids or in the vicinity of each other suggesting that there are certain areas of the proteins that are more susceptible to modification. One of these is the section of β-sheet between amino acids 85–99 in β-lactoglobulin where the sequence is located on the outside of the tertiary structure which would be more prone to chemical modification. While heat treatment resulted in increased modifications of cysteine, proline and total oxidation in both whey and cream fractions, homogenisation led to a decrease in cysteine oxidation for whey fractions but an increase in cysteine and total oxidations for cream fractions. These protein modifications may be caused by the structural changes that occur during milk homogenisation and heat treatment including the protein denaturation, the increased surface of the fat globules (because of the reduction in the size of the milk fat globules) and the absorbance of proteins on the milk fat globule. This work provides knowledge of where proteins are susceptible to modifications. By understanding the pressure and temperature effects and when they occur, we can modify treatment to reduce these unwanted protein modification side effects (Nunes and Tavares, Reference Nunes and Tavares2019). We found that homogenisation temperature as well as homogenisation pressure affects the degree of protein modification and recommend using low homogenisation pressures and temperatures.
Supplementary material
The supplementary material for this article can be found at https://doi.org/10.1017/S0022029923000122.
Acknowledgements
This work was supported by AgResearch Crown Research Institute SSIF funding (contract number A22713). We thank Dr Santanu Deb-Choudhury, Dr Jeffrey Plowman and Dr Stefan Clerens for proofreading the manuscript.