The obesity epidemic is becoming one of the most important public health problems in many parts of the world, as it is associated with an increased risk of multiple chronic diseases, including several of the major causes of death and disability in the developed world (diabetes, CVD, stroke, hypertension and certain cancers). A recent study has estimated a 30 % increase in the prevalence of obesity and a 130 % increase in the prevalence of severe obesity over the next two decades(Reference Finkelstein, Khavjou and Thompson1).
The aetiology of obesity is complex as it is a multifactorial condition in which genetic, environmental and interaction factors are involved(Reference Keith, Redden and Katzmarzyk2–Reference Gonzalez-Bulnes, Pallares and Ovilo4). Among the environmental signals, nutritional status is known to have a profound impact on the development of obesity, not only during the development of the obese phenotype but also at earlier stages. In fact, it has been hypothesised that a deficient nutritional environment during the critical period of perinatal development programmes whole-body energy homeostasis for optimal survival under nutritionally deficient conditions(Reference Barker5–Reference Symonds, Sebert and Burdge7). When prenatal adaptations are mismatched with the environment that the individual confronts later in life, they may lead to metabolic alterations(Reference Gonzalez-Bulnes and Ovilo8). This process is known as ‘prenatal/fetal programming’ or ‘developmental origins of health and disease’(Reference Burdge, Hanson and Slater-Jefferies9). Epigenetic processes are known to be involved in the mechanisms responsible for the programming of body weight (BW) homeostasis(Reference Hanson and Gluckman10, Reference Lavebratt, Almgren and Ekström11), in agreement with the thrifty epigenotype theory, which states that DNA sequence polymorphisms may play a minor role in the aetiology of obesity and type 2 diabetes and, instead, disease susceptibility could be predominantly determined by epigenetic variations(Reference Stöger12). Evidence points to appetite and energy homeostasis pathways as the main targets of programming processes. Interventional studies in animals, mainly carried out with murine models, have shown that undernutrition during perinatal development results in hyperphagic offspring(Reference Vickers, Breier and Cutfield13, Reference Desai, Gayle and Han14), predisposes to obesity and metabolic disorders later in life(Reference Ikenasio-Thorpe, Breier and Vickers15, Reference Krechowec, Vickers and Gertler16), and leads to alterations in the hypothalamic mRNA levels of several neuropeptides involved in appetite and metabolism regulation(Reference Plagemann, Waas and Harder17, Reference Orozco-Solís, Matos and Guzmán-Quevedo18).
Although considerable work has been carried out with laboratory animal models, the extrapolation of the results obtained from them to humans is challenged by marked differences in energy partitioning, allometry and metabolic rate, and thus large animal models such as sheep and pigs are needed(Reference Gillooly, Brown and West19, Reference Phelan and Rose20). Pig is a commonly used biomedical model for some diseases including the metabolic syndrome or obesity, because, in spite of being a litter-bearing species, it has many feeding, physiological, metabolic and behavioural similarities with human beings(Reference Spurlock and Gabler21). The availability of pig breeds with a high fat deposition trend and obese phenotype (fatty pigs) is a further advantage for the choice of adequate animal model resources. Among the fatty pigs, the Iberian breed has recently been shown to be a robust, amenable and reliable translational model for studies on obesity(Reference Torres-Rovira, Astiz and Caro22, Reference Torres-Rovira, Gonzalez-Añover and Astiz23). A recent work carried out by our group has shown the effects of undernutrition in pregnant Iberian dams on their metabolic status and on the prenatal and early postnatal development of their offspring(Reference Gonzalez-Bulnes, Ovilo and Lopez-Bote24). In this work, maternal nutritional restriction during the last two-thirds of gestation influenced offspring prenatal and postnatal growth and resulted in a sex-specific differential effect on early postnatal catch-up growth. Females born to diet-restricted mothers show the greatest potential for growth from birth to weaning.
The primary objective of the present study was to assess the effect of maternal energy restriction on fetal programming by using a large animal model with genetic predisposition for obesity and taking into account the influence of offspring sex on programming effects. For the accomplishment of such an objective, we analysed the consequences of undernutrition in Iberian sows during mid-late gestation on the body and organ development, metabolic state and hypothalamic transcriptional adaptations of the newborns in relation to the regulation of energy homeostasis, glucocorticoid metabolism and methylation. Moreover, long-lasting phenotypic effects of prenatal malnutrition were evaluated by measuring offspring growth and adiposity after weaning and in adulthood.
Materials and methods
Animals and experimental procedures
The present study was carried out at the facilities of the CIA Deheson del Encinar (Toledo, Spain), under a Project License from the INIA (Spanish National Research Institute for Agricultural and Food Technology) Scientific Ethic Committee. Animal manipulations were carried out according to the Spanish Policy for Animal Protection RD1201/05, which meets the European Union Directive 86/609 on the protection of animals used in experimentation.
The study included eighty-six experimental units: forty-one newborns, nine stillborns and thirty-six adult pigs born to twenty Iberian multiparous (two to four parities) sows from the herd of the Torbiscal strain, subjected to two different dietary treatments during the last two-thirds of gestation. The Torbiscal strain is one of the main Iberian pig strains belonging to an early conservation programme established in 1945 at ‘El Dehesón del Encinar’ (Oropesa, Toledo, Spain). It is a closed herd of Iberian pigs with complete genealogy available back to 1945. The twenty sows employed to generate the experimental animals were selected after the diagnosis of pregnancy on day 35 and assigned to one of two experimental groups (n 10) following simple randomisation procedures (computerised random numbers). After randomisation, a similar distribution of sows according to age, number of previous deliveries and BW was confirmed in the groups. From day 35 to delivery, the sows were housed in individual pens of 5·5 m2 and fed a standard grain-based diet fulfilling either their daily maintenance requirements for pregnancy (control group, n 10)(25) or only 50 % of such quantity (restricted group, n 10). The mean values of the diet were 89·8 % of DM, 15·1 % of crude protein, 2·8 % of fat and 12·89 MJ metabolisable energy/kg (3·08 Mcal metabolisable energy/kg). The animals were fed twice a day (in the morning and in the afternoon) and given ad libitum access to water.
At farrowing, two piglets (one male and one female when possible) from each sow were randomly selected for killing, taking measurements and tissue sampling for gene expression studies (n 41; nine females and twelve males born to the control sows; nine females and eleven males born to the restricted sows). The representativeness of the sampling procedure carried out was checked afterwards by comparing the BW means of the samples v. the whole litters corresponding to both the groups; no bias was detected. Moreover, all the stillborns that had died in the first 24 h of life (three from the control group and six from the restricted group) were also measured, but not sampled. In total, fifty piglets were studied at neonatal stage (nine females and fifteen males born to the control sows; twelve females and fourteen males born to the restricted sows).
The established sample size of about ten animals per treatment × sex group was similar to that employed in previous gene expression studies following maternal dietary interventions in rats(Reference Plagemann, Waas and Harder17, Reference Orozco-Solís, Matos and Guzmán-Quevedo18) and was estimated to have a power of 80 % for detecting 1·5-fold differences in gene expression (sd 0·4) with a two-sided 0·05 significance level test (http://www.stat.ubc.ca/~rollin/stats/ssize/n2.html).
Finally, another two piglets (one male and one female when possible) from each sow in each group (control and restricted) were randomly selected after weaning (28 d of age) for assessing possible differences in growth and adiposity after weaning and at maturity (from 28 to 210 d of age). Thus, a total of thirty-six littermates (ten males and eight females in each group) were moved to collective pens at the facilities of the INIA Animal Laboratory Unit (Madrid, Spain). The piglets were housed in a naturally ventilated barn with a space allowance of 3 m2/pig. The experiment began when the piglets were 28 d of age. The piglets were housed grouped by sex and maternal treatment (four groups) and fed a standard grain-based diet fulfilling their daily maintenance requirements from weaning to 4 months of age(25). Each barn was provided with ten individual troughs to allow all the animals in each pen to eat at the same time. The ingredients and proximate composition of the diets are given in Table 1. During the period of 28–120 d of age, the availability of feed was restricted to a previously calculated feeding programme corresponding approximately to 110 g/kg live weight0·75. All the pigs were given the same amount of feed, which was split into two meals (at 09.00 and 18.00 hours). Mean feed intake throughout this period was 0·97 kg/d. From 120 to 210 d of age, the pigs had ad libitum access to an obesogenic diet with a composition similar to the one described previously, but enriched with fat (63·3 g/kg per d) and with 10·7 MJ net energy/kg (Table 1) for allowing the expression of obesity. Individual BW at 28, 120 and 210 d of age and batch feed intake in the ad libitum feeding period were recorded. Average daily weight gain was calculated during two periods: from 28 (weaning) to 120 d of age (restricted feeding) and from 120 to 210 d of age (ad libitum feeding). Back-fat thickness (ultrasonically determined at the level of the head of the last right rib) was measured at 7 months of age. Throughout the experiment, the pigs had ad libitum access to water.
Table 1 Ingredients and proximate composition of the diets provided during the growing period
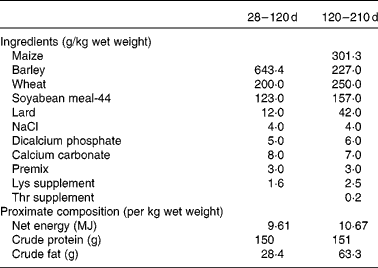
Evaluation of the metabolic status of the newborns
Blood samples were drawn from the forty-one selected newborns by puncture of vena cava cranialis and collected in 5 ml sterile heparin blood vacuum tubes (Vacutainer™ Systems Europe). Immediately after recovery, blood was centrifuged at 1500 g for 15 min, and plasma was separated and stored in polypropylene vials at − 20°C until assayed for the determination of the parameters of carbohydrate, protein and lipid metabolism and the concentrations of insulin, leptin, cortisol and corticosterone.
The concentrations of glucose, fructosamine, urea, TAG, total cholesterol, HDL-cholesterol and LDL-cholesterol were measured by means of a clinical chemistry analyser (Screen Point, Hospitex Diagnostics). The concentration of insulin was measured using a Porcine Insulin ELISA kit (Mercodia AB). The sensitivity of the assay was 0·26 UI/l (0·01 ng/ml); the intra-assay CV was 3·5 %. The concentration of leptin was determined in a single analysis using the Multi-species Leptin RIA kit (Demeditec Diagnostics GmbH). The sensitivity of the assay was 1·0 ng/ml; the intra-assay CV was 3·1 %. The plasma concentrations of cortisol and corticosterone were measured in a single analysis using ELISA kits (Demeditec Diagnostics GmbH). The sensitivities of the assays were 2·5 ng/ml and 1·6 nmol/l for cortisol and corticosterone, respectively; the intra-assay CV were 3·2 % for cortisol and 4·1 % for corticosterone.
Evaluation of the body, carcass and organ weights of the piglets
All the piglets born alive were weighed and measured at birth(Reference Gonzalez-Bulnes, Ovilo and Lopez-Bote24). For killing the forty-one live piglets, 2 ml of T-61 (MSD AH) were used. For all the stillborn and killed piglets (n 50), eviscerated carcasses and total viscera were weighed separately. Then, the individual weights of the brain, heart, liver, intestine, kidneys, loin (longissimus dorsi) and tenderloin (psoas major) were recorded.
For each piglet, the ratios of each organ weight:total BW were calculated.
Analysis of gene expression
At slaughter, the mediobasal hypothalamic region was dissected, snap-frozen in liquid N2 and stored at − 80°C until mRNA expression studies. Hypothalamic tissue samples were used to obtain total RNA using the RiboPureTM RNA isolation kit (Ambion) following the manufacturer's recommendations. The RNA that was obtained was quantified using the NanoDrop equipment (NanoDrop Technologies), and the quality of the RNA was assessed using an Agilent bioanalyser device (Agilent Technologies). The values of RNA integrity numbers obtained were in the range 8·0–9·0, thus assuring the homogeneity and high quality of the samples. First-strand complementary DNA synthesis was carried out using Superscript II (Invitrogen, Life Technologies) and random hexamers in a total volume of 20 μl containing 1 μg of total RNA following the supplier's instructions. Quantitative PCR was carried out for twenty candidate genes. Primers for amplification were designed from the available GENBANK and/or ENSEMBL sequences, covering different exons to ensure the amplification of the cDNA. Primer sequences and amplicon lengths are given in Table 2. Standard PCR for cDNA were carried out to verify amplicon sizes. The quantification of transcripts was done using SYBR Green Mix (Roche) in a LightCycler480 (Roche). Quantitative PCR mixtures were prepared in a total volume of 20 μl containing 2·5 μl of cDNA (1/20 dilution), 10 μl of SYBR Green Mix and 0·15 μm of both forward and reverse primers. As negative controls, mixes without cDNA were used. Cycling conditions were 95°C for 10 min, followed by forty-five cycles of 95°C (15 s) and 60°C (1 min) where the fluorescence was acquired. Finally, to test the specificity of the PCR, a dissociation curve was generated through one cycle at 95°C (15 s) followed by 60°C (20 s) and ramp up to 95°C with the acquired fluorescence during the ramp to 0·01°C/s. Data were analysed using the LightCycler480 SW1.5 software (Roche). All points and samples were run in triplets as technical replicates and dissociation curves were constructed for each individual replicate. Single peaks in the dissociation curves confirmed the specific amplification of the genes. For each gene, the efficiency of the PCR was estimated by standard curve calculation using four points of cDNA serial dilutions. Mean C p values were transformed to quantities using the comparative C p method, setting the highest relative quantities for each gene to 1 ($$quantity = 10^{ - \Delta C _{p}/slope} $$). The normalisation of quantitative PCR expression data was carried out using normalisation factors calculated with the Genorm software (http://medgen.ugent.be/~jvdesomp/genorm/) from the expression values of glyceraldehyde-3-phosphate dehydrogenase (GAPDH) and beta-2-microglobulin (B2M). Relative quantities were divided by the normalisation factors, which were the geometric means of the quantities of the two reference genes.
Table 2 Gene selection and primer design for RT-quantitative PCR
Statistical analyses
For the analysis of metabolic, weight and gene expression data, we used independent linear mixed models for each trait. The analyses were carried out using the SAS software (version 9.1, 2008, SAS Institute, Inc.). The model included the effects of treatment, sex and their interaction as fixed effects and a random litter effect accounting for the correlation among the records of full-sibs. Where significant, birth weight and the time elapsed since the deliveries to the tissue sampling were fitted as covariates in the gene expression data analyses. Significant differences were established using Tukey–Kramer honestly significant difference post hoc contrasts and were assumed to be statistically significant for P≤ 0·05.
Results
Body, carcass and organ weights of the newborns and stillborns
No differences were observed in the number of piglets born alive. Similarly, neonatal mortality was not significantly different between the groups (six stillborn piglets of the seventy-six born in the restricted group v. three of the eighty-three in the control group, P= 0·28). Body, carcass and organ weights were recorded for fifty animals, and the ratios of each organ weight:total BW were calculated (Table 3).
Table 3 Effects of maternal feed restriction during gestation and piglet sex on body and carcass weights (g) and on the ratios (g/kg) of each organ weight:body weight (BW) at birth (Mean values with their standard errors)
There was a significant effect of group (P <0·01) and sex (P <0·01) on BW and carcass weight at birth. The animals born to the restricted sows were lighter than their control counterparts, and females were lighter than the males.
The ratios of organ weight:BW were similar for both the groups (Table 3), except for the brain weight:BW ratio, which was significantly higher in the restricted group (P< 0·01). In fact, the absolute brain weight was similar between both the control and restricted animals (33·9 (sem 0·5) v. 34·1 (sem 0·5) g, respectively, P= 0·85), in spite of greater differences being observed in BW. The effect of sex was only significant for the tenderloin weight:BW ratio, which was higher in males than in the females (P <0·01).
No significant interaction (treatment × sex) effect was found either on the total weights or on the weight ratios.
Metabolic status of the newborns
The metabolic status of the newborns was assessed by the determination of the concentrations of leptin, insulin, cortisol and corticosterone and the parameters of carbohydrate, protein and lipid metabolism in the serum samples. The effect of maternal nutritional treatment and the treatment × sex interaction on the biochemical and hormonal status of the newborns is summarised in Table 4.
Table 4 Effects of maternal feed restriction during gestation and of the treatment×sex interaction on serum metabolic markers and hormone concentrations of newborn piglets (Mean values with their standard errors)
C, control; R, restricted; CF, control females; CM, control males; RF, restricted females; RM, restricted males.
a,b,c,dMean values within a row with unlike superscript letters were significantly different (P< 0·05).
No significant effect of dietary treatment was found for the concentrations of glucose, fructosamine and cholesterol. A significant difference between the groups was found for the concentration of TAG, which was 40 % lower in the restricted group (P <0·01). The concentration of urea was also slightly lower in the restricted animals, with a difference close to statistical significance being observed (P <0·07).
No significant effect was observed for the serum concentrations of leptin and insulin. The serum concentration of cortisol was significantly affected by the treatment, in a sex-dependent manner (P <0·04 for the interaction effect). Females born to the restricted sows exhibited the highest concentration of cortisol, while those born to the control sows exhibited the lowest concentration (Table 4). The concentrations of corticosterone were not significantly different between the restricted newborns and the control ones. No significant effect of sex was detected for any of these variables.
Hypothalamic gene expression in the newborns
Based on their putative involvement in prenatal programming processes (Table 2), twenty candidate genes were selected. Most of them were successfully amplified and quantified in the hypothalamic tissue samples of the piglets. Only three genes, 11β-hydroxysteroid dehydrogenase 2 (HSD11B2), suppressor of cytokine signalling 3 (SOCS3) and DNA methyl transferase 3B (DNMT3B), were not studied due to very low or absent amplification, indicating a low or null expression level.
Results are presented by grouping the genes with common biological functions (Fig. 1). For the candidate genes involved in the control of feed intake, a different response was observed for those genes involved in the transmission of the leptin anorexigenic signal (leptin receptor (LEPR) long isoform, insulin receptor (INSR), pro-opiomelanocortin (POMC), cocaine and amphetamine-related transcript (CART), signal transducer and activator of transcription 3 (STAT3) and melanocortin receptor 4 (MC4R)) or for the orexigenic genes with an opposite function (neuropeptide Y (NPY), agouti-related protein (AGRP), galanin (GAL), hypocretin (orexin) neuropeptide precursor (HCRT) and fat mass and obesity associated (FTO)).
Fig. 1 Relative gene expression, measured by quantitative PCR, in the hypothalamus of the newborns according to piglet sex and maternal feed restriction during gestation: control females (, n 9); control males (□, n 12); restricted females (
, n 9); restricted males (
, n 11). Results correspond to the selected candidate genes with (A) an anorexigenic role (LEPR, leptin receptor; INSR, insulin receptor; POMC, pro-opiomelanocortin; CART, cocaine and amphetamine-related transcript; STAT3, signal transducer and activator of transcription 3; MC4R, melanocortin receptor 4), (B) an orexigenic role (NPY, neuropeptide Y; AGRP, agouti-related protein; GAL, galanin; HCRT, hypocretin (orexin) neuropeptide precursor; FTO, fat mass and obesity associated), (C) a role related to glucocorticoid activity (GR, glucocorticoid receptor; HSD11B1, 11β-hydroxysteroid dehydrogenase 1; CRH, corticotropin-releasing hormone; TRH, thyrotropin-releasing hormone) and (D) a role related to DNA methylation (DNMT1, DNA methyl transferase 1; DNMT3A, DNA methyl transferase 3A). Values are means, with their standard errors represented by vertical bars. a,bMean values with unlike letters were significantly different (P <0·05 for the interaction effect).
The analysis of anorexigenic peptides indicated a statistically significant transcriptional response to maternal undernutrition for LEPR and POMC genes (P <0·02 and P <0·003, respectively, for the treatment effect). The restricted animals exhibited a lower expression of both the genes. Moreover, a significant interaction effect (treatment × sex) was observed for these two genes, with restricted females exhibiting a lower expression than the other groups (Fig. 1(A)). This lower expression in restricted females was statistically significant for LEPR and POMC (P <0·01 and P <0·04, respectively, for the interaction effect) and suggestive for MC4R (P <0·06 for the interaction effect).
On the other hand, the analysis of possible differences in the gene expression of orexigenic peptides indicated that the differences were not statistically significant (Fig. 1(B)).
For the genes involved in glucocorticoid function and stress (glucocorticoid receptor (GR), 11β-hydroxysteroid dehydrogenase 1 (HSD11B1), corticotropin-releasing hormone (CRH) and thyrotropin-releasing hormone (TRH)) a significant effect of treatment was observed for the expression of HSD11B1 (P <0·05), with restricted animals exhibiting a lower gene expression than the control ones (Fig. 1(C)). This effect seemed to be not affected by sex.
For the two analysed genes related to DNA methylation (DNA methyl transferase 1 (DNMT1) and DNA methyl transferase 3A (DNMT3A)), differences in expression observed were not statistically significant (Fig. 1(D)).
Body weight, weight gain and adiposity after weaning and in adulthood
BW measures were obtained from thirty-six descendants at weaning (28 d) and 120 and 210 d of age. Back-fat thickness was also recorded at 210 d of age, at the end of the trial. The records at 120 d of age coincide with the moment at which the diet was changed from a restricted to an ad libitum one, and thus these data allowed us to study the weight gain in the two feeding periods separately.
We did not detect any significant effect of maternal treatment or piglet sex on any of the post-weaning growth measures. Nevertheless, significant interaction effects (treatment × sex) were observed for BW at 120 and 210 d of age, for back-fat thickness at 210 d of age and for average daily weight gain in both the periods (Table 5). We did not detect significant differences in the weight of the four groups at 28 d of age in spite of greater differences being detected at birth. Based on the present results, it can be concluded that the restricted female group exhibited a higher growth rate than the control ones before and after weaning, resulting in higher BW, back-fat thickness and average daily weight gain values at 120 and 210 d of age, showing in general similar values compared with the control males. The difference in weight gain in the restricted females with respect to their control counterparts was similar during both the analysed periods (18 % during the restricted period and 14 % during the ad libitum period). During the ad libitum period, the values of mean feed intake were 3·2, 2·8, 3·6 and 2·8 kg/d for the control females, control males, restricted females and restricted males, respectively, which were within the normal range of values for feed intake for this breed. These feed intake values were calculated from batch data of each group, thus preventing their statistical analyses.
Table 5 Effects of the interaction of maternal undernutrition during gestation and piglet sex on post-weaning growth (Mean values with their standard errors)
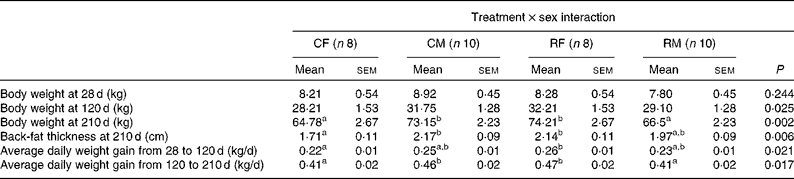
CF, control females; CM, control males; RF, restricted females; RM, restricted males.
a,bMean values within a row with unlike superscript letters were significantly different (P< 0·05).
In adulthood (7 months of age), females born to the restricted sows were the heaviest, followed by the control males, restricted males and control females (P< 0·002 for the interaction effect). The restricted females and control males had very similar values for back-fat thickness, followed by the restricted males and control females (P< 0·006 for the interaction effect). Adult females born to the restricted sows weighed 14 % more and had 25 % more back-fat thickness than their female control counterparts (P< 0·01 for both traits). In contrast, adult males born to the restricted sows were lighter (P< 0·04) than those born to the control ones, and the difference in back-fat thickness between both the male groups lacked statistical significance.
Discussion
Nutritional restriction during gestation predisposes to metabolic alterations in the offspring. Although the underlying mechanisms are not well understood, there is evidence of fetal compensatory adjustments, associated with metabolic and endocrine functions, playing a major role. The knowledge of molecular and cellular adaptations is critical to understand normal and abnormal development and physiology. We have previously shown the effects of undernutrition during pregnancy on the prenatal and early postnatal development of the offspring in sows with obesity/leptin resistance(Reference Gonzalez-Bulnes, Ovilo and Lopez-Bote24). In the present study, our goal was to test and examine the processes of prenatal programming in this robust large animal model, by studying the morphometry of the newborns of feed-restricted sows in mid-late gestation, their metabolic state and their hypothalamic gene expression patterns for key genes.
Body and organ weights and symmetry of the newborns
The present results indicate that birth BW was significantly affected by both the maternal diet and the offspring sex, with predictable results: restricted newborns were smaller than the control ones and females were smaller than the males(Reference Symonds, Sebert and Burdge7). Moreover, in the present study, the brain weight:BW ratio was significantly higher in the restricted newborns than in the control newborns. In fact, brain weight was similar in both the groups, in spite of greater BW differences being observed. This indicates that the effect of undernutrition was not proportional among all the body parts of the newborns, with the growth of the brain being less compromised. This asymmetrical growth restriction is known as the ‘brain-sparing effect’(Reference Giussani26, Reference Simmons, Gounis and Bangalore27) and seems to be characterised by a redistribution of the fetus cardiac output and preservation of blood flow to the carotid vessels in the uterus(Reference Al-Ghazali, Chita and Chapman28, Reference Veille and Cohen29), thus sparing the development of key organs at the expense of other organs. The ‘brain-sparing effect’ is known to be primarily caused by poor maternal nutrition, especially when the insult on fetal growth occurs late in the pregnancy, when the fetus becomes more nutritionally demanding, in accordance with the present experiment and results. The detailed morphometric study carried out in the present experiment confirms this asymmetric growth.
Metabolic profiling: hormones and parameters of carbohydrate, protein and lipid metabolism
The metabolic profiling of the piglets indicated some alterations induced by maternal undernutrition. A lower concentration of TAG was observed in the restricted animals in comparison with the control ones. This is probably a consequence of maternal hypotriacylglycerolaemia observed in the restricted sows(Reference Gonzalez-Bulnes, Ovilo and Lopez-Bote24). Maternal TAG are considered to be a major source of energy and fatty acids for the developing fetus(Reference Herrera30, Reference Herrera31), and thus low maternal and offspring TAG concentrations may be associated with a deficient development. Piglets born to the restricted sows also exhibited a trend for a lower plasma concentration of urea when compared with the control piglets, suggesting a lower protein turnover, in accordance with a thrifty metabolism.
Cortisol and corticosterone are the two main active physiological glucocorticoids in the serum. Fetal cortisol values exhibit a natural rise in late gestation, which has been linked to the maturation of several organs and systems in the process of preparation for delivery and postnatal life(Reference Seckl32). In conditions of maternal undernutrition, the induced maternal stress has been reported to lead to a significant increase in the levels of circulating endogenous glucocorticoids in both the mother and the fetus(Reference Lesage, Blondeau and Grino33, Reference Nijland, Mitsuya and Li34). Moreover, sex-specific effects on the hypothalamic–pituitary–adrenal axis and on the glucocorticoid levels of adult offspring following prenatal stress have been reported in rats(Reference Weinstock, Matlina and Maor35, Reference McCormick, Smythe and Sharma36), guinea pigs(Reference Lingas and Matthews37–Reference Schöpper, Palme and Ruf39) and primates(Reference Uno, Eisele and Sakai40). Excessive endogenous cortisol production is involved in retarded fetal growth(Reference Lesage, Blondeau and Grino33) and is considered to be one possible mechanism involved in offspring metabolic programming following prenatal environmental insults(Reference Belkacemi, Jelks and Chen41). The present results show that the concentration of cortisol increases significantly in female descendants of the restricted mothers, which have 2-fold higher cortisol concentrations than the remaining groups. The results are in agreement with a state of metabolic stress affecting the female descendants of the feed-restricted mothers. The differences observed in the concentration of cortisol in the offspring could be due to placental exchange, namely the impact of maternal serum levels, or to the intrinsic fetal characteristics. Unfortunately, we had no access to the serum samples of the pregnant sows at the end of pregnancy, which would have been useful to evaluate the contribution of maternal hormonal status to the differences observed in the concentration of cortisol in the newborns. Nevertheless, this limitation is partly compensated for by the inclusion of the maternal effect (random litter effect) in the statistical analyses. Moreover, the female-specific effect observed on the concentration of cortisol indicates an effect independent of maternal influences, which should equally affect both the sexes, unless a differential placental transfer according to the fetus sex had occurred.
Hypothalamic gene expression in the newborns and growth and fatness in adulthood
The Iberian pig breed is characterised by a great adipogenic trend and a phenotypic pattern of leptin resistance(Reference Morales, Pérez and Baucells42–Reference Gonzalez-Añover, Encinas and Gomez-Izquierdo45). The high voluntary feed intake observed in this breed despite elevated leptinaemia indicates a defect in leptin signalling. This defect is supposed to be related to a thrifty genotype(Reference Nunn, Bell and Guy46), secondary to polymorphisms in genes coding for energy balance regulation key proteins. Among them, a previously identified variant in porcine LEPR gene(Reference Ovilo, Fernández and Noguera47, Reference Ovilo, Fernández and Fernández48) has an allele fixed in Iberian pigs that results in an intense reduction of its hypothalamic expression, also affecting the expression of downstream genes and possibly leading to a reduction of the ability of leptin to induce a central anorexigenic signal. Thrifty genotypes have adapted over time to seasonal cycles of feasting and famine by fixing allelic variants that lead to efficient fat deposition during food abundance periods. The results of the present study indicate that this genetic predisposition for obesity may be further modulated by nutritional influences in prenatal periods, as maternal energy restriction seems to disturb the hypothalamic circuits that regulate energy homeostasis, reducing the expression of genes involved in the transmission of the leptin anorexigenic signal: the expression of LEPR and POMC genes was down-regulated in the restricted females and the same trend was observed for MC4R gene. The reduction in the expression of LEPR and POMC genes was intense as the restricted females exhibited approximately half the expression exhibited by their control counterparts. The limited number of animals studied may have affected our capacity to significantly detect other smaller effects, which could be very relevant from a biological point of view. The present results are in agreement with the results of studies in rodent models, in which maternal undernutrition programmes for a positive energy balance favouring the hypothalamic orexigenic pathways(Reference Ikenasio-Thorpe, Breier and Vickers15, Reference Krechowec, Vickers and Gertler16, Reference Breton49). The present results are also compatible with the results of studies in intra-uterine growth-restricted (IUGR) female piglets, in which a differential distribution of LEPR-expressing cells was observed among the hypothalamic nuclei and a lower expression of LEPR was observed in the arcuate nuclei of IUGR females(Reference Attig, Djiane and Gertler50), which are the main nuclei sampled in the present study.
Our previous work has established that nutritional restriction in Iberian sows during the last two-thirds of gestation results in a sex-specific differential effect on early postnatal catch-up growth, from birth to weaning(Reference Gonzalez-Bulnes, Ovilo and Lopez-Bote24). The weight of the restricted females, which was lowest at birth, reached that of the control counterparts by the time of weaning. Moreover, in the present study, the maternal undernutrition effect was also observed on the phenotype of older animals, from weaning to 7 months of age, with restricted females exhibiting the highest potential for growth and fattening. This sex-specific effect on weight gain and fattening is in agreement with the hypothalamic gene expression findings. Restricted females exhibit a change in hypothalamic gene expression compatible with a programming effect that leads to a reduction of the anorexigenic effects of leptin. Hyperphagic and anabolic trends may be thus expected in these animals, which could explain the differential growth and adiposity observed at early postnatal and later ages. In fact, higher voluntary feed intake is observed in the restricted female group during the ad libitum feeding period, but the present results indicate a similar response of a higher growth rate in the restricted females during the two post-weaning feeding periods (restricted and ad libitum), which supports the existence of metabolic adaptations towards a higher anabolism that are not dependent only on voluntary feed intake.
Sexual dimorphism in response to nutritional and environmental influences has recently been reported by human and animal studies(Reference Bellinger and Langley-Evans51–Reference Tang, Bi and Valego53) and has been reported to be related to a variety of factors including sex differences in the placental function and a different adaptability of placentas to environmental challenges depending on the fetus sex(Reference Clifton54). Moreover, transcriptomic and methylation studies also support this different placental functionality conditional on the fetus sex(Reference Mao, Zhang and Sieli55, Reference Gallou-Kabani, Gabory and Tost56). Recently, (Reference Wang, Pringle and Sykes57) the placental renin–angiotensin system has been shown to be regulated in a sex-specific manner. The genes of this system have been postulated to play key roles in placental angiogenesis(Reference Pringle, Tadros and Callister58), leading to the hypothesis of placental sex-specific functional differences giving rise to morphological differences in mechanisms as important as vascularisation.
Additional mechanisms mediating the effects of maternal undernutrition on fetal brain development may be secondary to the well-known detrimental effects of excess glucocorticoid exposure on the developing central nervous system(Reference Giussani26, Reference Seckl and Meaney59). In the present study, a significant increase in the plasma concentration of cortisol was observed in the restricted female newborns. Glucocorticoid signalling is mainly regulated by the HSD11B system. HSD11B type 1 mainly functions by converting cortisone and 11-dehydrocorticosterone to their biologically active forms cortisol and corticosterone, respectively. Conversely, HSD11B type 2 inactivates bioactive cortisol and corticosterone, acting as a glucocorticoid barrier. We observed a significant down-regulation of the expression of HSD11B1 in the restricted animals, which might result in a counter-regulatory mechanism aimed at reducing the activation of the inactive glucocorticoid precursors and thus limiting the negative effects of elevated concentrations of cortisol at the hypothalamic level. On the other hand, the elevated concentrations of cortisol observed in the females could be a consequence of sex-specific placental deficiency of the glucocorticoid-inactivating enzyme HSD11B2(Reference Lesage, Blondeau and Grino33, Reference Belkacemi, Jelks and Chen41, Reference Kapoor, Dunn and Kostaki60). These results support the prenatal programming effect observed in the restricted females being caused by excess glucocorticoid exposure, due to stress-induced glucocorticoid increase in feed-restricted mothers and offspring and probably due to a different glucocorticoid regulation at the placental level in both the sexes(Reference Clifton54). Moreover, the morphometric analysis carried out in the present study indicates that blood and nutrient supply to the brain is somewhat protected in the restricted animals, as the development of the brain seems to be not hampered in this group. Thus, we hypothesise that a direct effect of maternal nutritional restriction is less likely to play a main role in the determination of the hypothalamic programming effects, although it may probably play a major role in fetal growth restriction, as stated in previous works(Reference Belkacemi, Jelks and Chen41).
Maternal undernutrition is known to induce an overall loss of DNA methylation in CpG dinucleotides of different gene promoters involved in homeostasis and metabolism(Reference Nijland, Mitsuya and Li34, Reference Vo and Hardy61, Reference Lillycrop, Phillips and Torrens62), and variation in the expression or activity of DNMT1 has been postulated as a possible link between maternal diet and epigenetic regulation of gene expression in the fetus(Reference Burdge, Hanson and Slater-Jefferies9). Moreover, high stress-induced corticosteroid levels may alter one-carbon metabolism by reducing the availability of folic acid and could also be related to the modulation of DNMT1. Nevertheless, in the present study, no significant differences were detected in the expression of DNMT1 and DNMT3A genes.
Conclusion
To the best of our knowledge, there are no previous works that have been carried out in pigs genetically predisposed to obesity, addressing the physiological and transcriptomic outcomes of perturbations of the nutritional environment during the fetal period. The present results support a direct involvement of glucocorticoids, following nutritional metabolic stress, as determinants of prenatal programming effects, which are related to changes in the hypothalamic gene expression of neurotransmitters involved in energy balance regulation. The results of gene expression analyses are in accordance with the programming of the female descendants of undernourished Iberian sows for further positive energy balance. The effects observed are translated into growth and fattening changes in later postnatal ages, supporting the higher predisposition to obesity in the restricted females. A clear sex-specific effect was detected, which highlights the necessity for analysing the sex effect as a key factor in prenatal programming processes. These findings support the usefulness of the obese pig model employed for translational studies focused on the mechanisms and consequences of prenatal programming in human beings.
Acknowledgements
The authors thank the staff of the CIA Deheson del Encinar for their skilled technical assistance. They also thank A. I. Fernández, M. C. Rodríguez and M. A. Toro for revising the manuscript.
The present study was supported by funds from the Spanish Ministry of Economy and Competitiveness (project AGL2010-21 991-C03-02), co-funded by the FEDER. M. A. was given a grant by the Spanish Ministry of Economy and Competitiveness. The Spanish Ministry of Economy and Competitiveness had no role in the design and analysis of the study or in the writing of this article.
The authors' contributions are as follows: C. O., A. G.-B. and C. L.-B. conceived and designed the experiment; C. O., A. G.-B., R. B., M. A., A. B., M. L. P.-S., C. B. and S. A. carried out the experiment; A. F., C. O., R. B., M. A. and C. B. analysed the data; C. O., A. G.-B., A. B., M. L. P.-S., S. A. and A. F. contributed the reagents/materials/analysis tools; C. O. wrote the paper. All authors revised and approved the final version.
None of the authors has any conflicts of interest, financial or otherwise, to declare.