Introduction
Volcanic eruptions result in mineral-rich soils. As a result, soils at different locations may have different mineral compositions and physical and chemical properties. The presence of non-crystalline and crystalline clay minerals, such as allophane, imogolite, and halloysite, may result in differences in the soils. Clay minerals cover 0.84% of the earth's surface and are always found near active or inactive volcanoes.
Piedra Negras Series volcanic soil in southern Chile taken from the top 20 cm of the soil horizon was reported to contain allophane (Calabi-Floody et al., Reference Calabi-Floody, Bendall, Jara, Welland, Theng, Rumpel and Mora2011). Imogolite was found to form at a depth of 100 cm in the volcanic soil of the island of Réunion, Indian Ocean (Levard et al., Reference Levard, Doelsch, Basile-Doelsch, Abidin, Miche, Masion and Bottero2012). The mineralogical composition of volcanic soil taken from a depth of 25–105 cm at the southern foot of Mount Kilimanjaro indicates the presence of halloysite, smectite, and gibbsite (Van Ranst et al., Reference Van Ranst, Kips, Mbogoni, Mees, Dumon and Delvaux2020).
Indonesia has more volcanoes (>150) than any other country, scattered over almost every one of its islands (Simkin & Siebert, Reference Simkin and Siebert1994). One of the most active volcanoes is Mount Gamalama, located on Ternate Island, North Maluku province. Mount Gamalama has been active since 1538, leading to the creation of millions of tons of volcanic soil. Gamalama volcanic soil contains allophane with an irregular spherule shape, a diameter of 4 nm, a Si:Al ratio of 1.45, and a surface area of 125.158 m2/g (Cipta et al., Reference Cipta, Limatahu, Nur Abu, Kartini and Arryanto2017). No comprehensive study of clay minerals in Gamalama volcanic soil has been carried out previously. The identification and characterization of the allophane is the only study reporting the presence of nanoclay in Gamalama volcanic soil. The present study was conducted by taking soil samples from one location with a depth of less than 30 cm from the surface. Theoretically, allophane is a metastable precursor to halloysite formation and is associated with imogolite in volcanic soils (Takahashi et al., Reference Takahashi, Dahlgren, Theng, Whitton and Soma2001; Vacca et al., Reference Vacca, Adamo, Pigna and Violante2003).
Besides having significant agronomic potential with natural fertility, volcanic soils contain crystalline and non-crystalline clay minerals with dimensions of <2 μm (Delmelle et al., Reference Delmelle, Opfergelt, Cornelis and Ping2015). The formation of volcanic soils through weathering of volcanic glass parent materials including silicon, aluminum, and iron will form mineral products such as allophane, imogolite, or halloysite (Delmelle et al., Reference Delmelle, Opfergelt, Cornelis and Ping2015; Zehetner et al., Reference Zehetner, Miller and West2003). Allophane with a globular structure is non-crystalline with the chemical formula Al2O3SiO2·nH2O and a Si/Al ratio varying from 1 to 2 (Henmi & Wada, Reference Henmi and Wada1976). Imogolite has the chemical formula SiAl2O5·2H2O in a tube shape, 2–3 nm in diameter and several hundred micrometers long, and is associated with allophane in volcanic soils (Cradwick et al., Reference Cradwick, Farmer, Russel, Masson, Wada and Yoshinaga1972). Halloysite is a crystalline aluminosilicate clay mineral with the empirical formula Al2Si2O5(OH)4; it is tubular with a length of 1–2 μm, an outer diameter of 50–100 nm, and an inner diameter of 10–50 nm (Guimarães et al., Reference Guimarães, Enyashin, Seifert and Duarte2010; Hanif et al., Reference Hanif, Jabbar, Sharif, Abbas, Farooq and Aziz2016).
According to previous studies (Calabi-Floody et al., Reference Calabi-Floody, Bendall, Jara, Welland, Theng, Rumpel and Mora2011; Cipta et al., Reference Cipta, Limatahu, Nur Abu, Kartini and Arryanto2017; Levard et al., Reference Levard, Doelsch, Basile-Doelsch, Abidin, Miche, Masion and Bottero2012; Takahashi et al., Reference Takahashi, Dahlgren, Theng, Whitton and Soma2001; Vacca et al., Reference Vacca, Adamo, Pigna and Violante2003; Van Ranst et al., Reference Van Ranst, Kips, Mbogoni, Mees, Dumon and Delvaux2020), the Gamalama volcanic soil may also contain clay nanotubes, either halloysite or imogolite in a lower zone. The presence of halloysite or imogolite minerals in the Gamalama volcanic soil has never been studied or reported previously. The present study aimed to identify and characterize those nanotubes and other clay minerals in the volcanic soils of Mount Gamalama. The lack of information about nanoclay minerals in the Gamalama volcanic soil has meant that it is used only for plantation purposes. Information is provided here about the clay minerals in the soil and various possible nanotechnology applications are described. Applications in nanocomposite photocatalysts (Papoulis et al., Reference Papoulis, Komarneni, Nikolopoulou, Tsolis-Katagas, Panagiotaras, Kacandes and Katsuki2010), adsorbance (Ramadass et al., Reference Ramadass, Singh, Lakhi, Benzigar, Yang, Kim and Vinu2019), and in medicine (Hanif et al., Reference Hanif, Jabbar, Sharif, Abbas, Farooq and Aziz2016) may be possible.
Materials and Methods
Materials
The materials used in the present study were Gamalama volcanic soil, hydrogen peroxide (H2O2), sodium dithionite (Na2S2O4), sodium bicarbonate (NaHCO3), sodium citrate (Na3C6H5O7), and double-distilled water. The chemicals were of analytical grade from Merck, Darmstadt, Germany.
Methods
Volcanic Soil Sampling
Volcanic soils were collected from five locations at three depths, 30, 70, and 100 cm below the soil surface (Fig. 1). The sampling location coordinates of samples 1, 2, 3, 4, and 5 are (0°50’12.3" N, 127°19’18.6" E), (0°50’49.6" N, 127°19’43.2" E), (0° 49’59.5" N, 127°18’44.1"E), (0°49’54.0" N, 127°18’27.1" E), and (0°49’38.9" N, 127°18’09.0" E), respectively. The soil samples were first dried for several days to remove water and facilitate grinding. After drying, all samples were ground and sieved to pass a 200 mesh sieve. Each sample was labeled according to the sampling order and depth. For example, sample 1(70) means the soil sample was from a depth of 70 cm from the soil surface at location 1.

Fig. 1 Map of the locations of volcanic soil sampling at Gamalama Mt.
The soil-sampling depths and locations were selected based on the FAO/UNESCO revised classification of volcanic soil (Andosol). The definition of Andosol is based on the Andic and Vitric horizons that start from 25 cm from the soil surface. Andic horizons are of two primary types, silandic and alulandic. The andic horizon where allophane and similar minerals are predominant is called the silandic type. The alulandic type is regarded as a non-allophanic Andosol. These two major types are found between 30 and 100 cm from the soil surface (Takahashi & Shoji, Reference Takahashi and Shoji2001). Based on that theory, the Gamalama volcanic soils were taken from 30, 70, and 100 cm from the soil surface at five different locations in this study.
Separation of Clay Minerals
Volcanic soil samples from Mount Gamalama were first dried at room temperature for three days. A total of 50 g of dry soil sample was dispersed in a glass beaker using 300 mL of distilled water. The soil sample was stirred slowly using a magnetic stirrer and heated at 60°C. In the process of removing the organic content, 30% H2O2 was added to the sample. Stirring was stopped after the addition of 10 mL of 30% H2O2. After 24 h, the small floating particles <2 μm in size were separated slowly from the mixture using a pipette. The colloid particles were then subjected to treatment with DCB (Dithionite Citrate Bicarbonate) to remove the organic matter and some iron materials (Mehra & Jackson, Reference Mehra and Jackson1958). The solid was collected using a centrifuge at 4000 rpm (1790×g) for 30 min (at fixed angle with 6×20 mL rotor timer control). The solid sample was then washed using 30% H2O2 to remove organic anions that may have been adsorbed during the DCB treatment. About 5 g of solid was then dried at room temperature for 24 h and this was continued by heating in an oven at 60°C for 2 h.
Characterization of Clay Minerals
The characterizations were done using Fourier-transform infrared spectroscopy (FTIR, Shimadzu model 8201PC, Columbia, Maryland, USA), X-ray diffractometry (XRD, Shimadzu model 6000, Columbia, Maryland, USA). scanning electron microscopy (SEM, Hitachi model SU3500, Chatsworth, California, USA), and transmission electron microscopy (TEM, JEOL model JEM 1200, Tokyo, Japan). The chemical composition was evaluated by X-ray fluorescence (XRF, Bruker model S2 Puma Series 2, Karlsruhe, Germany) and surface area analysis (Micromeritics Tristar II 3020, Norcross, Georgia, USA).).
RESULTS AND DISCUSSION
Infrared Spectra
The FTIR spectra showed similarities in the absorption peaks of each sample (Fig. 2). For example, the spectra showed significant absorption at 3448, 1635, 1033, 910, and 532 cm–1. The peak at 3448 cm–1 indicated hydroxyl groups that may come from surface silanol, aluminol, or water (Bonelli et al., Reference Bonelli, Bottero, Ballarini, Passeri, Cavani and Garrone2009). The 1635 cm–1 band accompanying this peak is typical of bending vibrations of H2O (Frost et al., Reference Frost, Kristof, Horvath and Kloprogge2000). The Si–O–Si vibration was indicated by the absorption peak at 1033 cm–1 (Papoulis et al., Reference Papoulis, Komarneni, Nikolopoulou, Tsolis-Katagas, Panagiotaras, Kacandes and Katsuki2010; Yuan et al., Reference Yuan, Southon, Liu, Green, Hook, Antill and Kepert2008). The 910 cm–1 band showed the Si–O–Al stretch, characteristic of Al-allophane or aluminosilicate minerals (Henmi et al., Reference Henmi, Tange, Minagawa and Yoshinaga1981). The absorption peak at 532 cm–1 indicated Al-octahedra, originating from the halloysite gibbsite layer (Parfitt et al., Reference Parfitt, Furkert and Henmi1980). Finally, an absorption peak at 439 cm–1 indicated the bending vibration of Si–O–Si (Garcia-Valles et al., Reference Garcia-Valles, Alfonso, Martínez and Roca2020).

Fig. 2 FTIR spectra of Gamalama volcanic soils from five locations at three depths from the surface
The absorption pattern, especially in the 1100–900 cm–1 area, tended to be broader from samples from locations 1 to 5. This broadening effect was probably due to the hydrogen bonds in the sample related to the predominant clay. The presence of tubular clays, i.e. halloysite or imogolite, may increase the number of hydrogen bonds. Due to the tubular morphology, hydrogen bonding involving the outer-surface OH groups is likely (Tunega & Zaoui, Reference Tunega and Zaoui2020).
XRD Characterization
The XRD patterns revealed that halloysite exists in all of the samples tested, with various amounts of allophane and kaolinite, except for the sample from location 1 at a depth of 30 cm (Fig. 3, 1(30)). The XRD pattern of that sample showed a broad peak centered at 26.93°2θ (3.3 Å) belonging to allophane according to JCPDS No. 00-038-0449. The diffraction peaks shown by samples 1(70) and 1(100) at 8.26°2θ (10 Å), 19.69°2θ (4.5 Å), and 35.3°2θ (2.54 Å) are indicative of hydrated 10 Å-halloysite minerals, JCPDS No. 00-029-1489. Samples 1(70) and 1(100) showed a low-intensity peak of allophane, suggesting halloysite formation through allophane transformation by weathering of volcanic ash (Parfitt et al., Reference Parfitt, Russell and Orbell1983).
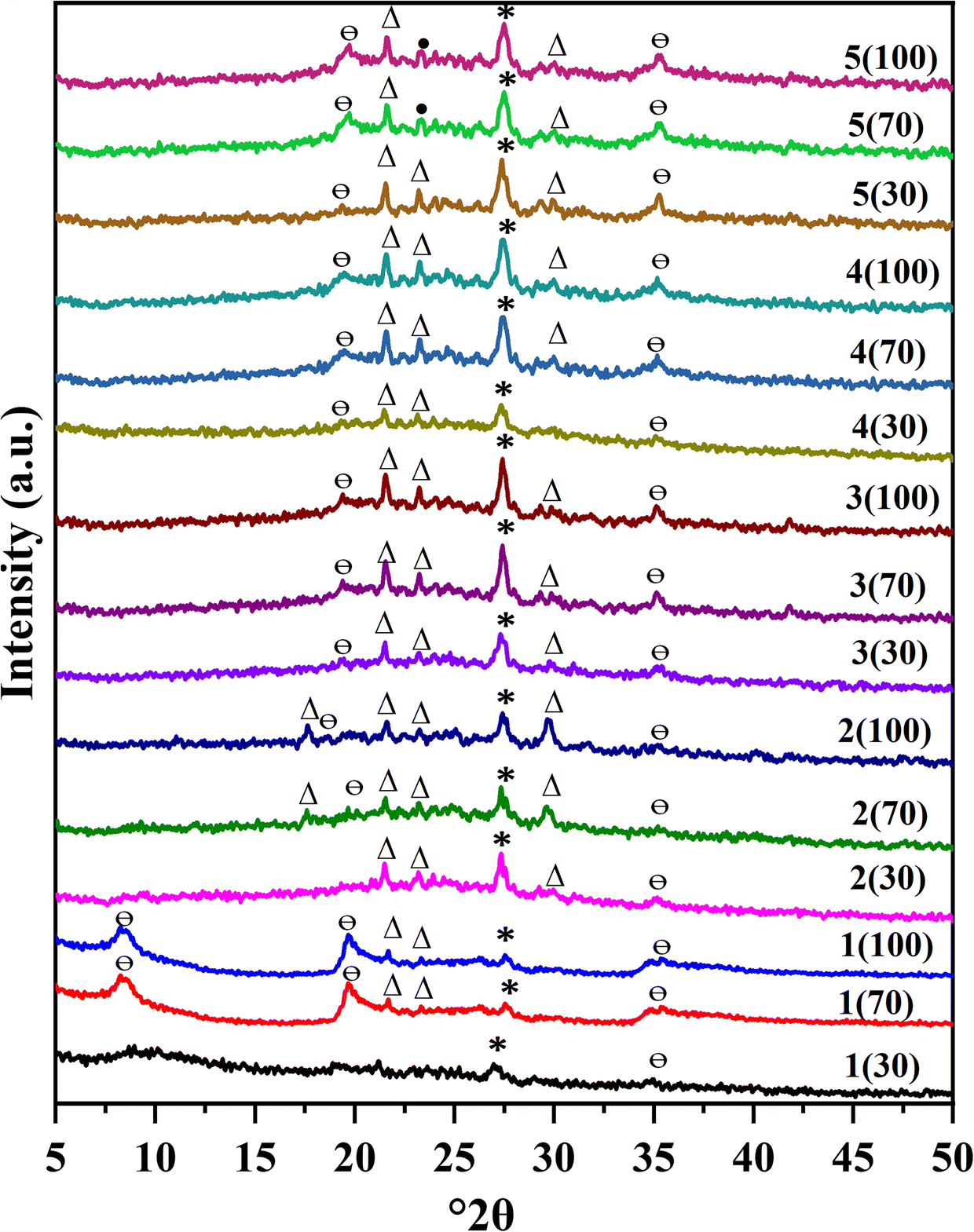
Fig. 3 XRD patterns of Gamalama volcanic soils from five locations at three depths from the surface. *: allophane; Θ: halloysite; ∆: kaolinite; •: metakaolinite
Samples at location 2 showed diffraction patterns dominated by kaolinite and less halloysite at increasing depth. Diffraction peaks at 21.6°2θ (4.12 Å), 23.13°2θ (3.84 Å), and 29.12°2θ (3.06 Å) correspond to the diffraction pattern data of kaolinite (JCPDS No. 01-078-2109). The halloysite diffraction peaks were observed at 19.6°2θ (4.5 Å) and 35.3°2θ (2.54 Å) for sample 2(70), according to reference JCPDS No. 00-029-1489. A diffraction peak at 27.3°2θ (3.26 Å) for sample 2 confirmed the allophane following the reference (JCPDS No. 00-038-0449).
Kaolinite minerals are present in samples 3 and 4 at various depths by the appearance of diffraction peaks at 21.6°2θ (4.12 Å), 23.13°2θ (3.84 Å), and 292θ (3.09 Å) according to JCPDS No. 01-078-2109, as well as allophane reference JCPDS No. 00-038-0449 and halloysite JCPDS No. 00-029-1489. The intensity of kaolinite and allophanes increased at deeper soil levels, while the halloysite remained constant.
Environmental conditions such as rainfall and Si concentration in the soil solution affect significantly the allophane transformation process in volcanic soils. The concentration of Si in the soil solution will decrease with increasing rainfall, indicating an increase in soil leaching (Parfitt et al., Reference Parfitt, Russell and Orbell1983). In conditions of low rainfall, the leaching process that occurs is low, causing the concentration of Si in the soil to be high (Harsh, Reference Harsh2005; Parfitt et al., Reference Parfitt, Russell and Orbell1983). According to the local Meteorology, Climatology, and Geophysical Agency, Ternate has low rainfall of ~1500 mm per year. With this paucity of rainfall, allophane tends to transform into halloysite in the Gamalama volcanic soil. Halloysite is a type of clay classified as kaolin and is usually developed early in the weathering process of volcanic soil. Halloysite is less stable than kaolinite and will transform into kaolinite over time (Huggett, Reference Huggett2005). The diffraction patterns in samples 3 and 4 indicated the presence of allophane, halloysite, and kaolinite. However, the kaolinite intensity increased with increasing depth. Deeper soil can be older, and the minerals in it undergo a longer weathering process. So, at increasing depth, the transformation of halloysite to kaolinite is more pronounced. Kaolinite was dominant at location 2, probably due to the longer soil formation and mineral-weathering processes than at other locations.
Samples 5 showed diffraction peaks at 19.71°2θ (4.50 Å) and 35.28°2θ (2.54 Å), indicating halloysite (JCPDS No. 00-029-1489). Allophane diffraction peaks were also observed in all samples 5, at 27.3°2θ with d-spacing 3.26 Å (JCPDS No. 00-038-0449), and kaolinite (JCPDS No. 01-078-2109) also. However, the intensity of allophanes tended to decrease with increasing sample depth. The minerals found in the 70 and 100 cm depth samples may have undergone a longer weathering process than those at 30 cm depth. Theoretically, weathering will cause allophane to transform into halloysite, imogolite, or kaolinite (Delmelle et al., Reference Delmelle, Opfergelt, Cornelis and Ping2015; Parfitt et al., Reference Parfitt, Russell and Orbell1983). The preferred transformation of allophane into halloysite occurs at a deeper location, however (Huggett, Reference Huggett2005).
Based on the XRD patterns, one can conclude that the Gamalama volcanic soil contains allophane, halloysite, and kaolinite. Different clay minerals dominate at each location. The differences are due probably to the high and low hydration processes involved during weathering, related to the chemical dissolution of the parent volcanic material. The weathering of volcanic materials generally dissolves Si, Al, and Fe. The rate and concentration of dissolution will affect the formation of secondary minerals such as allophane, halloysite, imogolite, or kaolinite (Delmelle et al., Reference Delmelle, Opfergelt, Cornelis and Ping2015). The process of soil hydration is related to the water content of the soil, which in turn is related to the height, slope, and amount of rainfall (Gusman et al., Reference Gusman, Nazki and Putra2018; Wahyuzi et al., Reference Wahyuzi, Zakaria and Sophian2018). The five locations have different slopes and distances from the coast, probably affecting the hydration process during weathering and causing the formation of the other minerals. However, this possibility still requires further study, such as identifying which clay minerals are present in the Gamalama volcanic soil at different heights and slopes.
SEM and TEM
Scanning (SEM) and transmission electron microscopy (TEM) were used to evaluate the morphology of the clay minerals. The mineral that dominates in each sample has a different morphology, as shown in Figs 4 and 5. Figure 4 shows spherules, stubby tubes, and plates of allophane, halloysite, and kaolinite (Bac et al., Reference Bac, Dung, Khang, Hung, Lam, An and Tinh2018; Levard et al., Reference Levard, Doelsch, Basile-Doelsch, Abidin, Miche, Masion and Bottero2012; Wang et al., Reference Wang, Cheng, Chai, Bian, Wang, Liu and Pan2020). Irregular spherules are dominant in sample 1(30), which aggregate to form a sphere diameter of ~0.1–0.5 μm. The shape of the irregular spherule is characteristic of allophane, confirming the XRD results of sample 1(30). Sample 1(70) (Fig. 4b) is dominated by the stubby porous tube shape, characteristic of halloysite. Samples 2(30) and 2(70) show the plate shape of kaolinite and irregular spherule of allophane, respectively. The kaolinite found in samples 2(30) and 2(70) has various sizes of between 1 and 3 μm. Likewise, allophane found in both samples has a variable length of between 0.1 and 0.5 μm. Samples 2(100), 4(30), 4(70), and 5(70) (Fig. 4e, f, g, h) showed the same morphological character, dominated by irregular spherules and tubes.
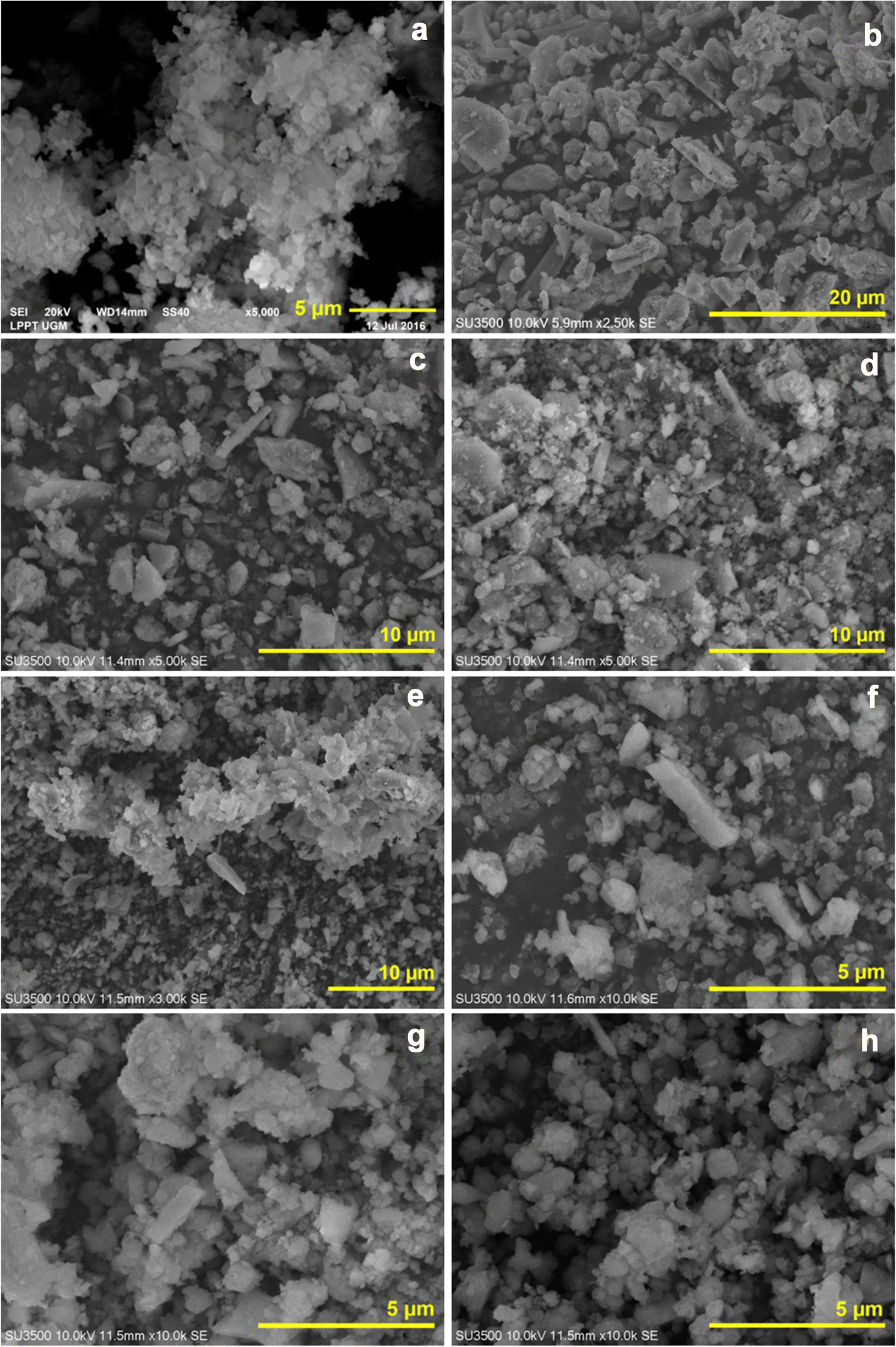
Fig. 4 SEM images of Gamalama volcanic soils: a 1(30), b 1(70), c 2(30), d 2(70), e 2(100), f 4(30), g 4(70), and h 5(70)
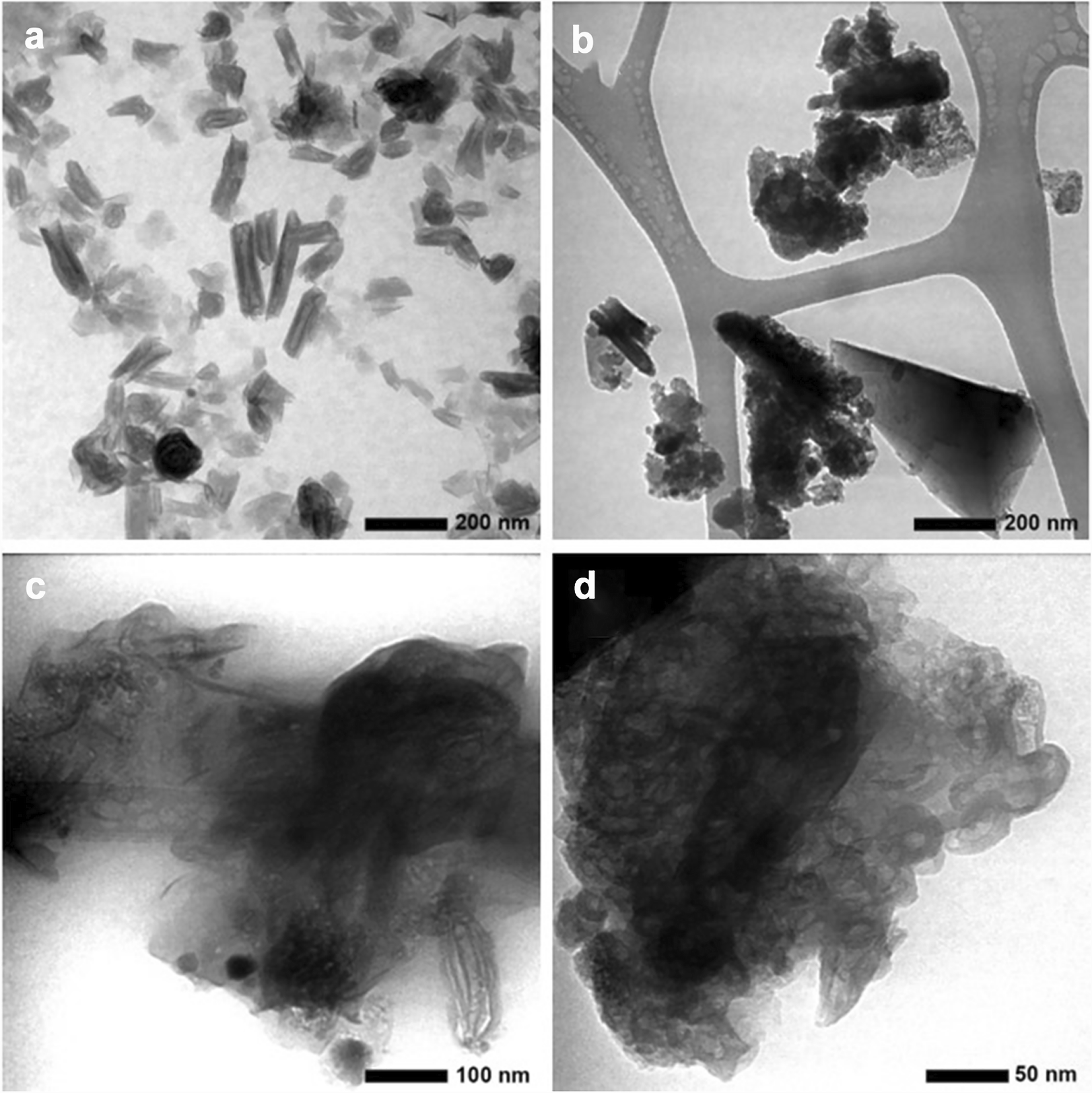
Fig. 5 TEM images of samples: a 1(70), b 2(70), c 4(70), and d 5(70)
Transmission electron microscopy images of samples taken from four different locations at the same depth from the surface, i.e. 70 cm, are shown in Fig. 5. The TEM images corroborated both the XRD (Fig. 3) and SEM results (Fig. 4). Sample 1(70) showed that the porous tubes of halloysite dominated with diameters of 10–20 nm and lengths of ~50–100 nm. The TEM image for sample 2(70) showed an aggregated spherical morphology with a diameter of 5–10 nm, a tube shape of ~20 nm diameter, and a length of ~100 nm. The shape of the plates, typical of kaolinite, was also observed with a size of ~400 nm in this sample. The TEM images from samples 4(70) and 5(70) also showed predominant stubby porous tubes with a diameter of 20 nm and a length of 50 nm but more aggregated. The TEM images indicated the presence of nanotube structures typical of halloysite and imogolite. The main difference between these two types of clay, apart from their XRD patterns, is the morphology. Halloysite is found naturally in spheroidal form with long, small, and stubby tubes. Natural imogolite is found in small, elongated tubes resembling fibers with a length of ~100 nm to several micrometers (Joussein et al., Reference Joussein, Petit, Churchman, Theng, Righi and Delvaux2005). Based on the TEM images, one can conclude that the predominant clay mineral in Gamalama volcanic soil is halloysite. Clay minerals with different structural and morphological characters in each Gamalama volcanic soil sample may affect the surface area and the pore volume.
XRF Characterization
The chemical composition of samples from four locations at 70 cm depth showed that silica and alumina are predominant with some Fe2O3 (Table 1). The molar ratio SiO2/Al2O3 of samples 1(70), 2(70), 4(70), and 5(70) are 1.35, 1.39, 1.46, and 1.35, respectively. The values indicate that the clay's dominant component is a 1:1 mixture, characteristic of halloysite and kaolinite (Bordeepong et al., Reference Bordeepong, Bhongsuwan, Pungrassami and Bhongsuwan2011). The SiO2/Al2O3 halloysite ratio in this study was similar to that found from several locations in Argentine Patagonia, which is 1.32–1.50 (Cravero et al., Reference Cravero, Fernández, Marfil, Sánchez, Maiza and Martínez2016). The existence of allophane with a Si/Al ratio >1–2 (Henmi & Wada, Reference Henmi and Wada1976) in all samples may also contribute to the higher SiO2/Al2O3 ratio. The chemical composition revealed a high Fe2O3 content in halloysite (15.49 to 18.04 wt.%), which is much greater than in some of the previously reported halloysites, i.e. 1.6% (Ben M’barek Jemaï et al., Reference Ben M'barek Jemaï, Sdiri, Errais, Duplay, Ben Saleh, Zagrarni and Bouaziz2015), 4.60% (Cravero et al., Reference Cravero, Fernández, Marfil, Sánchez, Maiza and Martínez2016), and 12.8% (Lutyński et al., Reference Lutyński, Sakiewicz and Lutyńska2019). The large Fe2O3 content found in the present study must have been the result of the high Fe content of the parent material from the Mount Gamalama eruption. Due to the significant Fe2O3 content, the halloysite found in Gamalama volcanic soil is probably ferro-halloysite (Ben M’barek Jemaï et al., Reference Ben M'barek Jemaï, Sdiri, Errais, Duplay, Ben Saleh, Zagrarni and Bouaziz2015).
Table 1 Chemical analysis of Gamalama volcanic soils (wt.%)

Specific Surface Area and Pore Size
The nitrogen adsorption-desorption method was used to determine the specific surface area and porosity. The adsorption isotherms (Fig. 6) of all selected samples were similar and followed types II and IV, indicating mesoporous material with large pore contribution. The hysteresis loop decreased with more kaolinite or allophane. The hysteresis loops may be due to the slit-shaped pores between cylindrical tubes, typically the smallest halloysite tubes. Sample 1(70) had the largest pore volume, while samples 2(70) and 4(30) were the smallest. The pore volume (Table 2) corresponded to the dominant clay phases in the sample. Samples containing predominantly halloysite showed large surface areas and pore volumes, whereas the presence of kaolinite, as detected in samples 2(70) and 4(30), resulted in small surface areas and pore volumes. The surface area was also closely related to the morphology of the material. The results of SEM and TEM images showed the predominance of stubby tube morphology in samples 1(70), 4(70), and 5(70), consistent with the large S BET value. As shown in SEM and TEM images of sample 2(70) (Figs 4 and 5), the existence of the plate-shaped kaolinite may significantly reduce the surface area and pore volume.
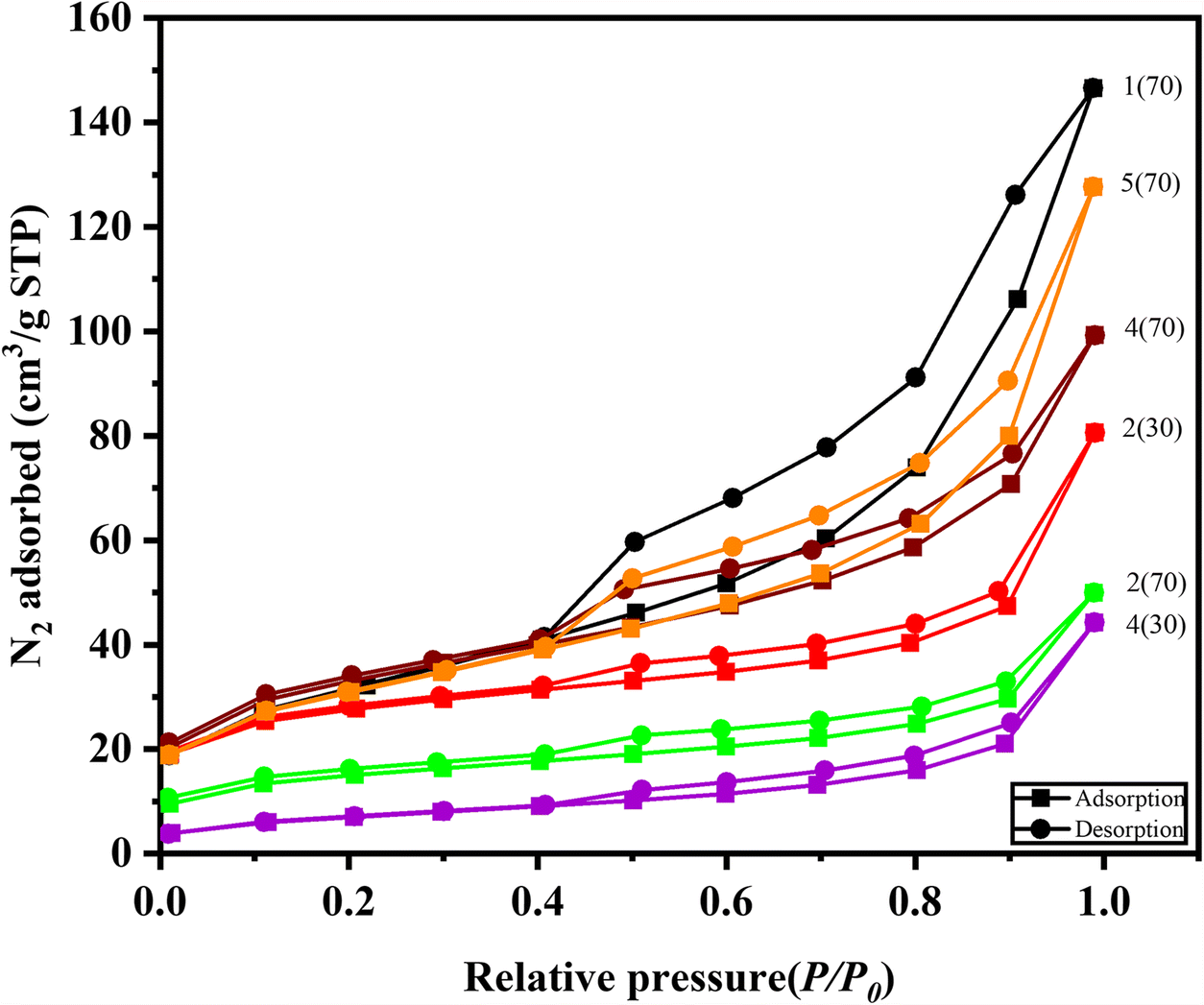
Fig. 6 Nitrogen adsorption/desorption isotherms of some samples of Gamalama volcanic soils
Table 2 S BET, pore size, and pore volume of the Gamalama volcanic soils

ǂ BJH cumulative pore volume (adsorption branch)
* Dominant phase identified by XRD (Fig. 3)
Table 2 shows that the morphology affects the surface area, thus influencing the effectiveness of the various applications of the clays (Dong et al., Reference Dong, Meng, Han, Zhao and Tang2019; Moriau et al., Reference Moriau, Bele, Marinko, Ruiz-Zepeda, Koderman Podboršek, Šala and Suhadolnik2021). The largest surface areas were observed in samples 4(70) and 1(70) at 112.510 and 110.737 m2/g, respectively. A tube-shaped halloysite dominates both samples. The surface areas of these two samples was larger than the halloysites originating from Australia, New Zealand, and the USA, which were reported to be 22–81 m2/g (Pasbakhsh et al., Reference Pasbakhsh, Churchman and Keeling2013). Sample 4(30) had the smallest surface area, 24.776 m2/g, with the smallest pore volume of 0.065 cm3/g. Likewise, sample 2(70) had a surface area of 50.371 m2/g.
The Gamalama volcanic soil with its halloysite content offers promise for possible use as adsorbents, supporting catalysts, or photocatalysts, due to its unique tubular structure and large surface area. Papoulis et al. (Reference Papoulis, Komarneni, Nikolopoulou, Tsolis-Katagas, Panagiotaras, Kacandes and Katsuki2010) revealed that natural halloysite with a surface area of 38 m2/g increased the photocatalytic activity of TiO2 by a factor of 3.74. Ramadass et al. (Reference Ramadass, Singh, Lakhi, Benzigar, Yang, Kim and Vinu2019) reported that natural halloysite with a surface area of 50.8 adsorbs as much as 6.17 mmol/g of CO2, more than commercial adsorbents of multi-walled carbon nanotubes (5.60 mmol/g) and mesoporous carbon nitride (5.30 mmol/g). The present authors predict that halloysites of samples 1(70) and 4(70) may result in a better performance due to their larger surface areas. Halloysite is also used as a drug carrier in drug-delivery systems due to its non-toxicity, biocompatible, high porosity, and good dispersion (Kamble et al., Reference Kamble, Ghag, Gaikawad and Panda2012). By using the simple method employed here, halloysite can be extracted from Gamalama volcanic soil as shown by samples 1(70) and 4(70). The remaining soil can be used for a variety of purposes, including as a geopolymer material, due to the large amounts of SiO2, Al2O3, and Fe2O3 (Zhou et al., Reference Zhou, Zhu, Lu and Li2022).
Conclusions
The clay minerals of the Gamalama volcanic soil from three depths at each of five locations were characterized and identified. Stubby halloysite tubes with a diameter of 10–20 nm and various lengths between 50 and 100 nm were found, especially at 70 cm. Irregular spherules of allophane and kaolinite plates were also observed. The surface area of the soil samples was between 24.7763 and 112.51 m2/g depending on the location. A large surface area was observed in samples that were dominated by halloysite. Applications for adsorption and supporting materials for catalysts or photocatalysts are envisaged for Gamalama volcanic soils.
Acknowledgments
The authors gratefully acknowledge financial support by a Universitas Gadjah Mada RTA Grant 2020.
Author Contribution
Conceptualization and final writing: Indriana Kartini
Writing – original draft preparation: Indra Cipta
Supervision: Indriana Kartini, Akhmad Syoufian
Data processing: Febiyanto
Funding
Universitas Gadjah Mada through RTA Grant 2020.
Data availability
The datasets generated during and/or analyzed during the current study are available from the corresponding author on reasonable request.
Declarations
Research Involving Human Participants and/or Animals
This study did not involve any human or animal participants.
Conflicts of interest
The authors declare that they have no conflict of interest concerning the study in this paper.