Nutritional immunology describes the influence of nutrition on the immune system, anti-viral activity and associated protective functions(Reference Wu, Lewis and Pae1,Reference Calder, Carr and Gombart2) . Deficiency in macronutrients and/or micronutrients causes impairment of immune function, which can be reversed by nutrient repletion. Among the nutrients that have important immunological roles and/or recovery from injuries/trauma, glutamine is probably the most widely recognised immunonutrient, as it is required for key immune-inflammatory responses. Glutamine plays various essential roles in almost every cell in the body, involving complex and dynamic regulations.
Although several amino acids play important roles in cell metabolism, glutamine is key for the intermediary metabolism and inter-organ nitrogen exchange via ammonia (NH3) transport between tissues. Glutamine can also be oxidised in the TCA cycle as an energy source, serve as a substrate for nucleotide synthesis, NAD+ and NADPH synthesis(Reference Curi, Newsholme and Marzuca-Nassr3,Reference Cruzat, Macedo Rogero and Noel Keane4) . In addition, glutamine has antioxidant properties by acting as a primary glutamate donor to the synthesis of γ-l-glutamyl-l-cysteinyl-glycine (also known as glutathione – GSH), and also chaperone properties by modulating the expression of heat shock proteins (HSPs)(Reference Cruzat, Macedo Rogero and Noel Keane4).
Glutamine is the most abundant amino acid in the blood, achieving concentrations of 2× to 100× greater than other amino acids with a concentration range of approximately 0⋅5–0⋅7 mm. Normal levels of glutamine in the body are usually achieved by a balanced diet associated with appropriate physical activity. However, it is already established that in catabolic situations, such as burns, post-trauma/surgery and exhaustive exercise, glutamine exogenous supply might be required due to the inability of the body to maintain optimal levels of the amino acid under some conditions (Fig. 1)(Reference Smedberg, Rooyackers and Norberg5). Glutamine may be required to attenuate immunodepression and help the body to recover more efficiently from an excess of damage and inflammation. Although glutamine exogenous supply seems a very plausible solution, it has always been a matter of vigorous debate. In this review, we aim to discuss the traditional and conceptual roles of glutamine, as well as its antioxidant and chaperone properties. We also describe an emergent glutamine role in brain physiology via the glutamate/gamma-amino butyric acid (GABA) cycle (GGC) in the context of exercise, and finally conclude with current considerations for translating possible glutamine supplementation effects for catabolic situations.
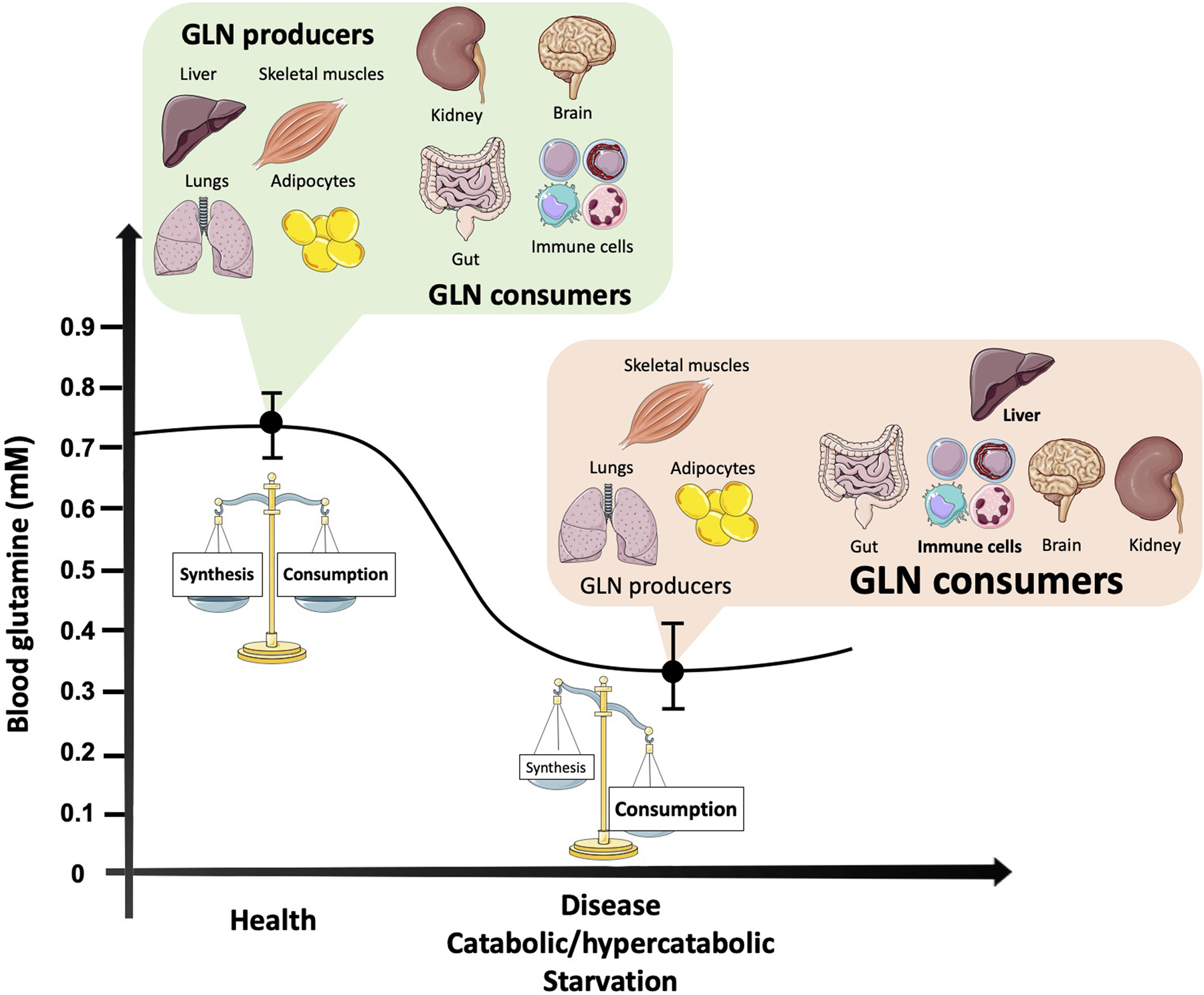
Fig. 1. Blood glutamine concentration changes according to the balance between major organ producers and consumers in health and catabolic situations. As the most abundant and versatile amino acid in the body, glutamine largely depends on the balance between its synthesis, release and uptake by organs and tissues. In turn, multiple intracellular pathways require glutamine as a substrate to maintain homoeostasis. In health, there is a balance between glutamine synthesis and degradation, while in catabolic situations organs responsible for glutamine synthesis reduce its production, such as the skeletal muscle tissue. Other glutamine producers, such as the adipose tissue and the lungs, do not have the capability to replenish the needs of the amino acid during catabolism. Moreover, the liver, a main glutamine producer in health becomes a major glutamine consumer under disease conditions, due to gluconeogenesis support. At the same time, cells of the immune system increase their demand for both glutamine and glucose. Although the brain and kidneys may have their glutamine capabilities altered according to the type of disease/catabolic condition, no significant changes may counteract the fall in glutamine (GLN) availability.
Brief overview of glutamine biochemistry and metabolism
Two principal enzymes regulate intracellular glutamine metabolism. Glutamine synthetase (GS; EC 6⋅3⋅1⋅2) catalyses the synthesis of glutamine from glutamate and ammonia in glutamine synthesising cells and tissues, while phosphate-dependent glutaminase (GLS; EC 3⋅5⋅1⋅2) catalyses the hydrolysis of glutamine to glutamate (with the release of ammonia). Glutamine availability largely depends on the balance between its synthesis, release and uptake by organs and tissues. The lungs, liver, brain, adipose tissue and skeletal muscles are the major glutamine suppliers as they exhibit high activity of tissue-specific glutamine synthesis, i.e. GS. In contrast, through the activity of GLS and other enzymes capable of degrading glutamine, the amino acid can undergo various rates of degradation. Cells of the intestinal mucosa, renal tubule and especially leucocytes are the predominant glutamine-consuming tissues. Under stress and/or catabolic conditions such as cancer, sepsis/infections, post-surgery and trauma, the endogenous synthesis of glutamine does not appear to meet the human body demand, and hence glutamine assumes the role of a conditionally essential amino acid(Reference Liu, Ma and Luo6–Reference Altman, Stine and Dang8). The blood glutamine concentration can fall by up to 30–50 % under catabolic conditions, while at the same time, skeletal muscle glutamine concentration may fall by up to 50 %(Reference Smedberg, Rooyackers and Norberg5,Reference Cruzat, Pantaleao and Donato7,Reference Cruzat, Bittencourt and Scomazzon9) .
Glutamine has a γ-amide nitrogen that is essential for the biosynthesis of nucleotides and other metabolites such as hexosamine. In nucleotide biosynthesis, glutamine and glutamate either directly or indirectly, via aspartate for example, serve as the nitrogen donors for all nitrogen atoms in purines and pyrimidines(Reference Chowdhry, Zanca and Rajkumar10). For rapidly dividing cells such as tumour cells, enterocytes and lymphocytes, glutamine consumption may be related to an urgent need for nucleotide biosynthesis(Reference Curi, Lagranha and Doi11). Glutamine is also used for the synthesis of GSH, the major endogenous antioxidant molecule in the cell. Cells are exposed to oxidative stress under conditions such as nutrient starvation (or oversupply) and catabolic stress such as surgery, sepsis or infection, but also during cell proliferation(Reference Cruzat, Pantaleao and Donato7,Reference Cruzat, Keane and Scheinpflug12) . The antioxidant properties mediated by glutamine are discussed later in this review.
Glutamine is an important nitrogen donor for the production of NAD+, in the last steps of both the de novo (from dietary tryptophan) and Preiss–Handler (from dietary niacin) pathways(Reference Matsuyama, Yoshinaga and Shibue13). NAD+ is an essential coenzyme and electron acceptor in catabolic pathways of metabolism(Reference Ryu, Nandu and Kim14). NAD+ is also produced through salvage pathways from nicotinamide and nicotinamide riboside precursors, however, people with deficiency or low activity of the GS gene exhibit severe secondary NAD+ deficiency(Reference Hu, Ibrahim and Stucki15), indicating that the glutamine supply is indispensable for NAD+ synthesis(Reference Wojcik, Seidle and Bieganowski16).
It is widely accepted that immune cell intermediary metabolism is critical to cell function. Glucose is mainly converted into lactate (glycolysis), whereas glutamine is converted into glutamate, aspartate and alanine by undergoing partial oxidation in the TCA cycle to carbon dioxide, aspartate, alanine and lactate in a process called glutaminolysis(Reference Curi, Newsholme and Marzuca-Nassr3). Furthermore, through the pentose phosphate pathway, cells can produce ribose-5-phosphate (a five-carbon sugar), which is a precursor for the pentose sugars required for synthesis of RNA and DNA, as well as glycerol-3-phosphate for phospholipid synthesis. The degradation of glutamine, via GLS action, yields ammonia (from the amide nitrogen of glutamine) for the ATP-dependent formation of carbamoylphosphate and also the formation of aspartate through glutaminolysis which ultimately leads to the synthesis of purines and pyrimidines required for DNA and RNA synthesis(Reference Wojcik, Seidle and Bieganowski16,Reference Mills, Kelly and O'Neill17) .
Glutamine and monocyte/macrophage cell function
Macrophages are associated with high consumption rates of glutamine and in addition, glutamine is essential for many of the functions of these leucocytes. The supplementation or in vitro treatment with glutamine optimises the function of these cells(Reference Cruzat, Macedo Rogero and Noel Keane4). Macrophages are diffusely scattered in the connective tissue and in liver (where they are termed Kupffer cells), spleen and lymph nodes (sinus histiocytes), lungs (alveolar macrophages) and central nervous system (CNS) (microglia). The half-life of the macrophage precursor, the blood monocytes, is about 1 day, whereas the life span of tissue macrophages is several months or years. The mononuclear phagocyte system, consisting of a number of cell types including monocytes and macrophages, is part of both humoral and cell-mediated immunity and has an important role in defence against microorganisms, including mycobacteria, fungi, bacteria, protozoa and viruses.
With respect to the mononuclear phagocytes, different populations of macrophages have now been identified, in vivo, including M1 and M2 macrophages(Reference Martinez, Sica and Mantovani18). M1-like macrophages are responsible for secreting pro-inflammatory cytokines and lipid mediators and are involved in tissue degradation and T lymphocyte activation as part of an inflammatory immune response(Reference Gordon and Taylor19). M2 macrophages exert different functions, such as contribution to tissue repair and the secretion of anti-inflammatory cytokines and alternative lipid mediators. Lipopolysaccharides (LPS) exposure in vitro promotes a switch from glucose-dependent oxidative phosphorylation to aerobic glycolysis in macrophages – typical of the Warburg effect(Reference Gordon and Martinez20). Pyruvate kinase M2 regulates hypoxia-inducible factor 1-α activity, a rapid increase in ATP formation and IL-1β expression, thus being a key regulator of the Warburg effect in LPS-activated macrophages(Reference Palsson-McDermott, Curtis and Goel21,Reference O'Neill and Pearce22) . M1 macrophages (treated with LPS) have two points of substrate flux deviation with regards to the TCA cycle (in contrast to M2 macrophages) one occurring at the isocitrate dehydrogenase step and another post-succinate formation. This is an important metabolic reprogramming mechanism. As a result, there is an accumulation of TCA cycle intermediates (e.g. succinate, α-ketoglutarate, citrate and itaconate) that impacts the activation of LPS-stimulated macrophages(Reference Harber, de Goede and Verberk23,Reference Tannahill, Curtis and Adamik24) . Itaconate, a recently discovered metabolic regulator in macrophages has anti-inflammatory properties through activation of nuclear factor erythroid 2-related factor 2 Kelch-like (ECH)-associated protein 1 is a protein that in humans is encoded by the Keap1 gene. Keap1 has been shown to interact with Nrf2, a key regulator of antioxidant responses, required for the amelioration of oxidative stress alkylation(Reference Mills, Kelly and O'Neill17). In contrast, Liu et al.(Reference Liu, Wang and Li25) reported α-ketoglutarate, generated through glutaminolysis (glutamine → glutamate → α-ketoglutarate), promotes M2 macrophage differentiation. Macrophage metabolism varies with specific-tissue microenvironment, and this metabolic reprogramming is required for macrophage differentiation and function.
Macrophages can produce relatively large amounts of nitric oxide, especially the M1 inflammatory macrophage, via increased concentrations of the enzyme inducible nitric oxide synthase, which may be stimulated by LPS or other immune activator exposure. The activated macrophage can actually release the enzyme arginase to deplete local concentrations of arginine (indeed arginine is required for tumour growth). It has been reported that mouse peritoneal resident and Bacillus Calmette–Guerin-activated macrophages and human monocytes are capable of utilising glutamine at high rates, contain sufficient activity of the enzymes required to convert glutamine to citrulline (and subsequently citrulline to arginine via enzymes of a partial urea cycle) to account for observed rates of nitrite synthesis (which in turn is derived from derived from NO) in the absence of extracellular l-arginine, and will release nitrite when exposed to intermediates of the proposed glutamine → arginine pathway(Reference Murphy and Newsholme26). Thus, the M1 macrophage can release arginase to deplete local concentrations of arginine but ensures it has sufficient intracellular arginine via biosynthesis from glutamine.
Glutamine, the glutathione axis and heat shock protein responses
An important aspect to be considered under stress and/or catabolic conditions is the redox state of the cell, as it plays an essential role in cell fate. This can be obtained from the ratio between the intracellular concentration of glutathione disulphide (GSSG) and GSH(Reference Cruzat, Keane and Scheinpflug12). The ratio is normally expressed as [GSSG]/[GSH], and in an oxidative environment there is a resulting reduction of GSH and an increase in GSSG(Reference Desideri, Ciccarone and Ciriolo27). The inhibition of GSH recycling from GSSG does not appear to impact GSH content and cell survival. Conversely, the inhibition of the de novo synthesis of GSH dampens intracellular antioxidant defence mediated by this system and increases oxidative stress(Reference Lian, Gnanaprakasam and Wang28). The initial step for the de novo synthesis of GSH occurs through the activity of glutamate-cysteine ligase, a heterodimer of a catalytic subunit and a modifier subunit that catalyses the first and rate-limiting step to form the dipeptide γ-glutamylcysteine from cysteine and glutamate(Reference Desideri, Ciccarone and Ciriolo27).
Glutamine via glutaminolysis can increase the substrate availability for the de novo synthesis of GSH(Reference Cruzat, Bittencourt and Scomazzon9). Numerous studies in animal models submitted to infection and trauma(Reference Cruzat, Pantaleao and Donato7,Reference Cruzat, Bittencourt and Scomazzon9) , as well as critically ill and post-surgery(Reference Wang, Wu and Zheng29) patients, have demonstrated that exogenous administration of glutamine (both enteral and parenteral nutrition), significantly enhances the GSH system, increasing cellular resistance to lesions and reducing oxidative stress(Reference Cruzat, Macedo Rogero and Noel Keane4,Reference Smedberg and Wernerman30) . Interestingly, similar effects have been reported in animal models submitted to exercise. For example, chronic oral administration with glutamine effectively increased glutamine availability in tissues, such as the liver and muscle, which amplified GSH stores and improved the redox state of the cell. These and other immune-inflammatory effects, such as lowering plasma cytokines, were observed in animal models submitted to swimming(Reference Cruzat, Rogero and Tirapegui31,Reference Cruzat and Tirapegui32) , treadmill(Reference Petry, Cruzat and Heck33,Reference Petry, Cruzat and Heck34) and resistance exercise(Reference Raizel, Leite and Hypolito35,Reference Leite, Raizel and Hypolito36) .
Whether glutamine availability is maintained by its exogenous supply or endogenously synthesised, this amino acid has unique metabolic and anti-inflammatory roles. One of the main anti-inflammatory properties mediated by glutamine is through the modulation of proteins with chaperone function, such as the HSPs(Reference Leite, Cruzat and Krause37). HSPs are classified into six families (i.e. HSP10, HSP40, HSP60, HSP70, HSP90 and HSP100) on the basis of their monomeric molecular weight. Almost all HSPs prevent the aggregation of newly synthesised polypeptide chains during folding, and some HSPs, such as HSP70 have also the ability to clear improperly folded and unfolded proteins. Under several stress conditions, HSPs are primarily regulated by transcriptional activators, such as the heat shock factors (HSFs)(Reference Martinez, Dias and Natov38). HSFs undergo trimerisation and translocate to the nucleus, where they bind heat shock elements within the promoter regions of their target genes, and activate the transcription of HSPs(Reference Dimauro, Mercatelli and Caporossi39). In addition to their role as molecular chaperons, HSPs are also able to associate with the complex formed by NF-κB with its inhibitor, and block NF-κB translocation to the nucleus. Hence, the HSP response has anti-inflammatory properties by virtue of turning NF-κB off and attenuating the production of inflammatory mediators. Glutamine enhances the transactivation of HSF1 and induces HSF1 expression via cytosine-cytosine-adenosine-adenosine-thymidine (CCAAT) box motif which is present in several gene promoters enhancer-binding protein-β in a dependent manner. Furthermore, glutamine induces HSP expression via N-acetyl-O-glycosylation and phosphorylation of HSF1 and Sp1 transcription factors. This pathway is coordinated by a special and nutrient-sensing pathway, the hexosamine biosynthetic pathway(Reference Sun, Shang and Yao40,Reference Tan, Sim and Long41) . Many in vitro (Reference Cruzat, Keane and Scheinpflug12), and in vivo experiments(Reference Moura, Lollo and Morato42), including animal models submitted to exhaustive exercise training(Reference Petry, Cruzat and Heck33,Reference Petry, Cruzat and Heck34) have identified the important role of glutamine availability/metabolism and the anti-inflammatory response mediated by HSPs. Interestingly, other glutamine coupling amino acids, such as arginine have shown to stimulate HSP effects in exercised rats(Reference Moura, Lollo and Morato42).
Glutamine, the brain and exercise
Glutamine is abundantly expressed throughout the mammalian CNS, with concentrations ranging from 5 nmol/mg in the hippocampus to 7 nmol/mg in the cerebellum(Reference Takado, Sato and Kanbe43). Glutamine within the CNS functions to regulate neurotransmission through the metabolism of the neurotransmitters, glutamate and GABA(Reference Schousboe44).
Glutamate and GABA are the major excitatory and inhibitory neurotransmitters expressed within the brain and function in concert to coordinate a plethora of physiological functions from memory formation to motivation and from respiration to appetite control(Reference Xu, Bartolome and Kong45). Within the mammalian brain, evolution has shaped several brain-glutamine intrinsic ‘innovations’ which are fundamental to the efficacy and predominance of glutamate and GABA neurotransmission. The blood–brain barrier tightly regulates brain extracellular composition of glutamine, glutamate and GABA irrespective of the level in the blood(Reference Hawkins and Vina46,Reference Dolgodilina, Imobersteg and Laczko47) . As the blood–brain barrier is relativity impermeable, the brain is capable of autonomous productions of key neurotransmitters(Reference Hawkins and Vina46). Glutamatergic/GABAergic synapses are encompassed by astrocytes with excitatory amino-acid transporters and high affinity GABA transporter 1 and 3 (Fig. 2)(Reference Leenaars, Drinkenburg and Nolten48). These transporters are capable of rapidly removing glutamate and GABA from synaptic clefts post-depolarisation in order to dramatically increasing the signal-to-noise ratio of neurotransmission(Reference Boddum, Jensen and Magloire49,Reference Leke and Schousboe50) . Glutamate and GABA neurotransmitter homoeostasis is tightly regulated via glutamine through the glutamine-GGC (Fig. 2)(Reference Leenaars, Drinkenburg and Nolten48). Here, glutamine is compartmentalised not within neurons but in neighbouring astrocytes. Astrocytes exclusively express GS hence inherently limiting glutamine production to astrocytes(Reference Castegna and Menga51). This unique feature of astrocytic glutamine pooling is critical to the spatial and temporal resolution of glutamate and GABA neuronal transmission(Reference Albrecht and Zielinska52).
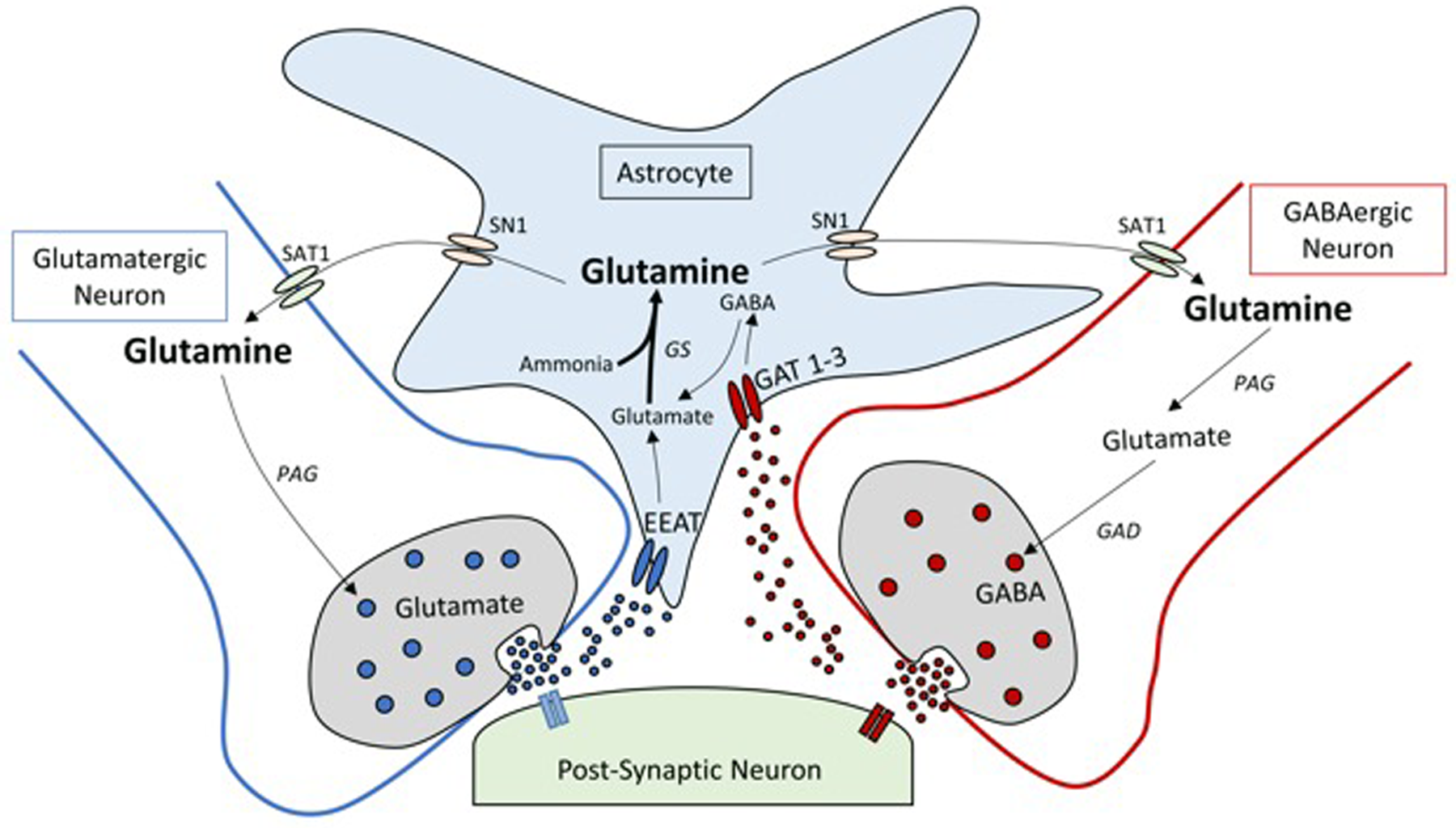
Fig. 2. Simplified schematic diagram of the glutamine-glutamate/GABA cycle (GGC). Within a glutamatergic and GABAergic neuron, glutamate (excitatory neurotransmitter) and GABA (inhibitory neurotransmitter) are released into the synaptic cleft. Following transmission, glutamate and GABA are taken up by excitatory amino-acid transporters (EAAT) and GABA transporter (GAT) 1 and 3 located in membranes of astrocytes. Glutamate taken up by the astrocytes is quickly aminated by glutamine synthetase (GS) to glutamine, whereas GABA is converted to glutamine via the tricarboxylic acid cycle and then to glutamine by GS. Glutamine exits astrocytes through the system N transporter 1 (SN1) transporter proteins to the extracellular space and diffuses to the surface of glutamatergic neurons, where it is taken up by diamine acetyltransferase (SAT1). Within the pre-synaptic neuron, glutamine is converted by phosphate activated glutaminase (PAG) back to glutamate or further converted to GABA by glutamic acid decarboxylase (GAD).
To replenish glutamine back to neurons, astrocytes shuttle glutamine through system N 1 transporter to diffuse across the extracellular matrix to the surface of presynaptic neurons. Here, glutamine it is taken up by diamine acetyltransferase (SAT1) and metabolised back to glutamate and GABA by the action of GLS and glutamic acid decarboxylase, respectively(Reference Verkhratsky, Nedergaard and Hertz53). Through the regulation of glutamate and GABA, glutamine within the CNS plays an integral role in many aspects of neurobiology and as such disturbances in glutamine metabolism and/or transport have been implicated in a range of pathologies ranging from chronic stress, epilepsy, hepatic encephalopathy, manganese encephalopathy, neurodegenerative disorders and metabolic disease(Reference Huang, Liu and Yin54,Reference Ivens, Caliskan and Papageorgiou55) .
A particularly unique feature of the GGC within the brain is that it is metabolically expensive. The clearance of glutamate by neurons and astrocytes from the synapse involves the co- and counter-transport of Na+ and K+ via metabolically demanding Na+–K+ pumps during neurotransmission(Reference Herbst and Holloway56). Further metabolic expenses is required for the subsequent formation of glutamine via GS in astrocytes as this is an ATP-dependent process(Reference Herbst and Holloway56). 13C-magnetic resonance spectroscopy kinetic studies tracing found that 60–80 % of resting energy utilised by both the human or rat brain is used to recycling glutamate and GABA between synapses and presynaptic terminals through the GGC(Reference Rothman, Behar and Hyder57). At rest the adult brain consumes about 20 % of the body's RMR (44–87 % during infancy, childhood and adolescence) despite accounting for only 2 % of body mass(Reference Kuzawa and Blair58). As such, GGC could account for as much as about 16 % of adult RMR indicating that glutamine metabolism is vulnerable during periods of metabolic activity such as exercise.
An emerging body of evidence suggests that physical exercise has a profound influence on the CNS. Pre-clinical and human studies demonstrate that exercise, whether that be acute or chronic, regulates neuronal activity particularly in regions such as the hippocampus, hypothalamus, cerebellum, basal ganglia and motor cortex to influence the neurophysiology underlying memory, neurogenesis, motivation and appetite(Reference Seo59–Reference Rodrigues, Pereira and de Campos63).
Unlike whole-body energy balance (balance between energy intake and expenditure), global brain energy balance is not altered during low-intensity exercise or during the transition from rest to exercise, however, increased metabolic rate is detectable at the whole-brain level during vigorous exercise(Reference Smith and Ainslie64,Reference Coxon, Cash and Hendrikse65) . Maddock et al. reported that vigorous exercise in human subjects increases glutamine levels within the cortex, an effect that occurs as a consequence of activity-dependent increases in the glutamate–glutamine turnover(Reference Maddock, Casazza and Buonocore66). Furthermore, involuntary exercise to exhaustion in rats, increased glutamine in the hippocampus, striatum and cerebellum(Reference Swiatkiewicz, Fiedorowicz and Orzel67). As exercise has been shown to attenuate the elevated central glutamate transmission in models of addiction and cardiovascular functions, it remains unclear if elevated levels of glutamine are a cause or consequence to these changes in brain function. It is well established that exhaustive exercise increases circulating NH3(Reference Weiner and Verlander68), and one possible explanation for the enhanced glutamine within the brain could be to detoxify the brain via the removal of NH3 through the synthesis of glutamine using GS(Reference Conway and Hutson69). Interestingly, branched chain amino acids (i.e. leucine, isoleucine, valine), glutamine and arginine may hypothetically enhance exercise performance and decrease serotonin, a mediator involved in central fatigue(Reference Conway and Hutson69). However, there is lack of in vivo evidence demonstrating that amino acid supplementation may effectively reduce central fatigue. The relative contribution of glutamine to the enhanced performance reported in relatively few studies has not yet been confirmed thus care must be taken with any glutamine supplementation exercise particularly as elevated GS levels are described to be elevated in rodent models of diet-induced obesity(Reference Soontornniyomkij, Kesby and Soontornniyomkij70) and other pathological conditions(Reference Huyghe, Denninger and Voss71). In addition, it is well known that high levels of NH3 induced by high amino acid intake have toxic effects, especially for the brain and the kidneys(Reference Conway and Hutson69,Reference Davani-Davari, Karimzadeh and Sagheb72) . Although there is an emerging role of central glutamine metabolism in exercise, the relative contributions of the GGC and its molecular machinery (GS, system N 1, SAT1, GLS and glutamic acid decarboxylase) to enhance exercise performance, including exercise-induced regulation of neuronal function and/or central fatigue remains unclear.
Glutamine supplementation
The relationship between glutamine metabolism (plasma and tissue), supplementation and immune-inflammatory responses have been investigated for more than 35 years(Reference Curi, Newsholme and Marzuca-Nassr3,Reference Cruzat, Pantaleao and Donato7) . The literature demonstrates an overall consensus regarding the fall of glutamine in critically ill patients, and this has been driving studies that provide glutamine exogenous supply. However, in situations where catabolism is more dynamic and/or less severe, which include exercise situations, glutamine levels in the body may fluctuate, and hence results from research might be conflicting and/or lacking in the literature (Fig. 1). For instance, Borgenvik et al.(Reference Borgenvik, Nordin and Mikael Mattsson73) observed a reduction in plasma concentrations of essential amino acids and glutamine during an adventure racing exercise, whereas no changes were detected in muscle concentration after exercise. Similar findings were also reported by Tritto et al.(Reference Tritto, Amano and De Cillo74) where plasma glutamine was unchanged and not associated with immunosuppressive responses in combat athletes undergoing to a rapid weight loss.
It is worth emphasising that, although plasma glutamine level is reduced from its normal concentration (i.e. 500–800 μmol/l) to 300–400 μmol/l, cells depending on this amino acid, are negatively impacted in terms of proliferation and function by this decrease(Reference Cruzat, Krause and Newsholme75). Exhaustive exercise can dramatically increase the catabolism of amino acids in the skeletal muscles and liver, which eventually might reduce the concentration of glutamine in plasma and associated tissues. However, this is more likely to be a gluconeogenic transitory situation, as glutamine provides nitrogen atoms to the synthesis of purines, pyrimidines and amino sugars. If the high glutamine degradation persists, many metabolic pathways and mechanisms that depend on glutamine availability can be affected and increase the potential of immunosuppression. Indeed, several studies have reported that immunosuppression induced by exercise excess can increase the risk of infections and the development or aggravation of overtraining syndrome(Reference Gleeson, Pyne and Elkington76,Reference Edouard, Junge and Sorg77) . However, the link between glutamine availability and infection seems to be clearer in critically ill patients(Reference Smedberg and Wernerman30) and less likely to play a mechanistic role in exercise-induced immunodepression(Reference Ramezani Ahmadi, Rayyani and Bahreini78).
Scientific evidence has shown the important immune-inflammatory roles of glutamine availability and/or glutamine metabolism in stressful and/or catabolic situations. However, several questions and specific considerations were raised in regards to quantity/dose, frequency and form of administration of l-glutamine supplementation. l-Glutamine is often supplied as a component of clinical nutrition supplementation pre- and post-operative surgery or in critically ill patients. In these situations, l-glutamine is usually administered by utilising its free form (also known as an isolated amino acid), or bond with another amino acid, also known as the dipeptide form, such as l-alanyl-l-glutamine or l-glycine-l-glutamine. In general, many clinical and experimental studies have shown more beneficial results when glutamine was administered as an intravenous solution, when compared to enteral routes. For instance, intravenous l-glutamine administration appears to reduce the rate of infectious complications, length of hospital stay and mortality of critically ill patients(Reference Smedberg and Wernerman30,Reference Stehle, Ellger and Kojic79,Reference McRae80) . It is important to note that these results are more likely to be achieved and/or be less heterogenous among patients and studies where intravenous or parenteral l-glutamine is provided to individuals with or without hypoglutaminaemia and receiving adequate energetic and protein supply (e.g. according to ESPEN recommendations(Reference Cruzat, Macedo Rogero and Noel Keane4,Reference Smedberg and Wernerman30) ). Conversely, intravenous or parenteral routes are always invasive, and it cannot be applied for sport and exercise situations. If l-glutamine supplementation is eventually recommended for elite athletes, oral routes are the best choice, although it is well known that rapidly dividing cells, such as the enterocytes, are extremely avid consumers of the amino acid. Glutamine is absorbed in the upper part of the small intestine and subsequently metabolised in the liver, thus it may not be available in sufficient quantity for other tissue targets, such as immune and muscle cells, to have a measurable effect.
An alternative way to bypass the high glutamine consumption of enterocytes is oral supplementation with glutamine dipeptides. Bonded amino acids (e.g. di- and tripeptides) have a differentiated membrane transport in the luminal membrane through the glycopeptide transport protein-1(Reference Boudry, Rome and Perrier81). This system allows a higher proportion of di- and tripeptides to escape hydrolysis and metabolisation be the enterocytes, and hence are more efficiently transported into the bloodstream and other tissues, such as the liver, immune system, kidneys and skeletal muscles. Interestingly, when free l-glutamine and free l-alanine are administered orally in combination, similar immune inflammatory effects were observed in animal models submitted to exhaustive exercise(Reference Cruzat and Tirapegui32). Although the precise mechanisms are still unknown, l-glutamine and l-alanine work in parallel, i.e. l-alanine is rapidly metabolised via alanine aminotransferase to pyruvate, with concomitant production of glutamate from 2-oxoglutarate, which contribute to antioxidant defence, such as GSH production. Hence, the presence of l-alanine in the l-alanyl-l-glutamine dipeptide or in its free form and supplemented in tandem, can spare glutamine metabolism in order for the latter to be used by high-demand tissues under inflammatory and/or highly catabolic situations(Reference Cruzat, Pantaleao and Donato7,Reference Cruzat and Tirapegui32) . This promotes the idea that l-glutamine supplementation should be accompanied by another amino acids or possibly proteins. Indeed, when l-glutamine was administered with proteins, such as wheat protein, or branched chain amino acids the whole-body glutamine status was increased(Reference Harris, Hoffman and Allsopp82) and muscle atrophy was more effectively inhibited(Reference Haba, Fujimura and Oyama83). Moreover, other amino acids, such as arginine may provide additional effects upon defence mechanisms, including the HSP response(Reference Moura, Lollo and Morato42). If the efficacy of l-glutamine supplementation is dramatically impacted by the presence or absence of other amino acids metabolised by the enterocytes, this would possibly explain, at least in part controversial results obtained in human subjects and animal models supplemented with l-glutamine and submitted to exercise. Other reasons for heterogeneous results include the quantity/dose and frequency of l-glutamine supplementation. At least in experimental models with rodents, both factors can significantly impact the possible immune and antioxidant effects mediated by l-glutamine supplementation.
Overall, current data indicate that glutamine supplementation may provide important metabolic and immunological support in catabolic situations, including critical care and elite athletes. However, as reported by other authors(Reference Smedberg and Wernerman30), several precise scientific questions, hypothesis and mechanistic explanations about the effects of glutamine exogenous supply were never tested in critically ill patients, nor in sport and exercise. For instance, should hypoglutaminaemia and/or tissue glutamine stores be the main factor driving the decision of supply of exogenous glutamine? Also, in catabolic situations that hypoglutaminaemia is not observed, can individuals obtain benefits, such as immune and antioxidant effects from glutamine supplementation?
Conclusion
The scientific literature continues to provide evidence of the importance of glutamine metabolism in cell function and homoeostasis, especially via heat shock responses and the GSH system. This is particularly important for cells of the immune system, such as monocytes/macrophages to maintain a continuous defence against microorganisms. Conversely, several questions remain about the efficacy of l-glutamine supplementation in catabolic situations, such as critical care and extenuating exercise. Possibly, the lack of consensus is related to the fact that quantity/dose and frequency of l-glutamine supplementation, as well as the presence or absence of other amino acids metabolised by the enterocytes significantly impact the desired amino acid supplementation outcomes. In the CNS for example, while disturbances in glutamine metabolism have been implicated in a range of neurodegenerative disorders, as glutamine is vital for glutamate and GABA concentrations, high levels of NH3 induced by high amino acid intake may have toxic effects. Although in vitro and animal studies continue to be important for the understanding of cell metabolism and physiology, more studies in human subjects are required to better elucidate the action and positive benefits of glutamine supplementation. The following suggestions may aid in deciding the formulation of future studies in immunometabolism:
• Determination of the role of glutamine metabolism and exogenous supply of glutamine-derived metabolic intermediates in the essential antiviral activity of immune cells.
• Glutamine supplementation and potential slowing of immunosenescence by altering immune cell metabolism and function.
• Mechanistic studies on enteral absorption of glutamine supplements, including concomitant amino acid administration to optimise glutamine immune-metabolic effects.
Acknowledgements
We acknowledge the Faculty of Health, Torrens University Australia for research support. We also acknowledge the Curtin Medical School and The Curtin Health Innovation Research Institute for research support and provision of excellent research facilities. G. T. D. also acknowledge The University of Melbourne for provision of research facilities.
Financial Support
This study was supported by Australian Research Council (ARC) Linkage grant LP190100130, National Health and Medical Research Council (NHMRC) of Australia (grants: 1160043, 2002427), Diabetes Australia Trust (grant: Y20G-DODG) and the Australian Research Council for research funding (grant: DP220102132) and the São Paulo Research Foundation (FAPESP), grant no. 2021/13119-2.
Conflict of Interest
The authors declare no conflicting or competing interests.
Authorship
P. N. and V. C. were responsible for the review outline and decisions regarding content of the review. V. C. prepared figures associated with this review. All authors contributed to writing of the text.