Introduction
Hydrogen is considered to be one of the most important energy carriers due to its high energy density and environmental friendliness. Currently, most of the hydrogen are still produced from fossil fuels. Although electrochemical water splitting is believed to be a promising alternative approach for hydrogen production, its practical applications are strongly limited by the lack of cheap and highly efficient electrocatalysts for oxygen evolution reaction (OER) and hydrogen evolution reaction (HER) [Reference Anantharaj, Ede, Sakthikumar, Karthick, Mishra and Kundu1]. The mostly commonly used electrocatalysts for HER are noble metal-based materials, such as Pt/C. To lower the device prices, there have been great efforts for the synthesis of noble metal-free electrocatalysts, such as transition metal sulfides [Reference Yang, Yao, Yu, Islam, He, Yuan, Yue, Xu, Hao, Sun, Li, Ma, Zapol and Kanatzidis2, Reference Lin, Wang, Wang, Li, Si, Qi, Cao, Zhong, Fei and Feng3, Reference Zhang, Wang, Zhang, Zhu, Zhang, Zhang, Ren, Song, Wang, Ying, Wang, Qiu, Peng and Fu4, Reference Wu, Liu, Han, Song, Shi, Song, Niu, Xie, Cai, Wu, Kang, Zhou, Chen, Zheng, Xiao and Wang5], oxides [Reference Xiao, Wang, Huang, Wei, Dong, Ma, Shen, Li and Wang6, Reference Jin, Wang, Su, Wei, Pang and Wang7, Reference Zhu, Liu, Jin, Chen, Lee, Liu and Chen8, Reference Zhu, Zhang, Zhao, Chen, Liu, Zhao, Wang, Liu, Chen and Liu9, Reference Liu, Zhang, Zheng, Guo, Zhu, Chen, Li, Liu, Yu, Wang, Liu, Chen and Liu10], and carbides [Reference Zang, Niu, Wu, Zheng, Cai, Ye, Xie, Liu, Zhou, Zhu, Liu, Wang and Qian11]. However, their performances still need to be further enhanced for competing with noble metal-based electrocatalysts for practical applications.
Due to its earth-abundant composition, low cost, and high activity, molybdenum disulfide (MoS2) has been reported to be a promising candidate to substitute noble metals as high-performance HER electrocatalysts. To further improve the HER performance of MoS2, many strategies have been investigated, such as the defects [Reference Chen, Huang, Ji, Adepalli, Yin, Ling, Wang, Xue, Dresselhaus, Kong and Yildiz12, Reference He, Zhao, Chen, Chen, Zhu, Su, Huang, Xue, Dai, Cheng, Liu, Wang and Chen13, Reference Li, Qin, Ries, Hong, Michel, Yang, Salameh, Bechelany, Miele, Kaplan, Chhowalla and Voiry14, Reference Tsai, Li, Park, Park, Han, Norskov, Zheng and Abild-Pedersen15] and strain engineering [Reference Li, Tsai, Koh, Cai, Contryman, Fragapane, Zhao, Han, Manoharan, Abild-Pedersen, Norskov and Zheng16, Reference Li, Du, Mleczko, Koh, Nishi, Pop, Bard and Zheng17], element doping [Reference Xiao, Liu, Zhang, Song, Feng, Gao and Ding18, Reference Liu, Zhu, Zhang, Xi, Tao, Gao and Xue19, Reference Luo, Ouyang, Zhang, Xiao, Ge, Jiang, Wang, Tang, Cao, Liu and Xing20, Reference Sun, Wang, Chi, Lin, Chen, Ling, Qiu, Xu, Song, Chen and Su21], morphology optimization [Reference Kong, Wang, Cha, Pasta, Koski, Yao and Cui22, Reference Kibsgaard, Chen, Reinecke and Jaramillo23], and phase control [Reference Wang, Zhang, Li, Zhang, Du, Yin, Bi and Yang24, Reference Yin, Han, Zhang, Zhang, Xu, Yuan, Samad, Wang, Wang, Zhang, Zhang, Cao, Song and Jin25]. Recently, heterointerface engineering has emerged as an effective approach to enhance the HER activity of MoS2 [Reference Ibupoto, Tahira, Tang, Liu, Morante, Fahlman, Arbiol, Vagin and Vomiero26, Reference Khalil, Liu, Muhammad, Habib, Khan, He, Fang, Masood, Rehman, Xiang, Wu and Song27]. For instance, Ibupoto et al. [Reference Ibupoto, Tahira, Tang, Liu, Morante, Fahlman, Arbiol, Vagin and Vomiero26] reported a composite nanostructure MoSx@NiO as an advanced nonprecious catalyst for HER in alkaline media, with an overpotential of 406 mV to produce 10 mA/cm2 current density and the lowest Tafel slope of 43 mV per decade. Lin et al. [Reference Lin, Wang, Wang, Li, Si, Qi, Cao, Zhong, Fei and Feng3] constructed defect-rich heterogeneous MoS2/NiSx nanosheets for overall water splitting. The interfaces of MoS2/NiSx with disordered structure presented excellent electrocatalytic performance with low overpotentials of 62 mV and 278 mV at the current density of 10 mA/cm2 for HER and OER, respectively. Yang et al. [Reference Yang, Yao, Yu, Islam, He, Yuan, Yue, Xu, Hao, Sun, Li, Ma, Zapol and Kanatzidis2] designed a hierarchical nano-assemble composite MoS2/Co9S8/Ni3S2/Ni with controllable composition and abundant interfaces as a highly efficient electrocatalyst for overall water splitting in a wide pH range. This hierarchical structure displays ultralow overpotentials (113, 103, and 117 mV for HER and 166, 228, and 405 mV for OER) at the current density of 10 mA/cm2 in alkaline, acidic, and neutral electrolytes, respectively.
As mentioned above, there have been many successful examples to construct MoS2-based heterostructures through one-step synthesis such as a solvothermal method [Reference Yang, Yao, Yu, Islam, He, Yuan, Yue, Xu, Hao, Sun, Li, Ma, Zapol and Kanatzidis2, Reference Li, Chen, Wen, Gui and Fang28, Reference Zhang, Wang, Pohl, Rellinghaus, Dong, Liu, Zhuang and Feng29]. However, obtaining heterostructures with a well-controlled microstructure and heterointerface properties remains a challenging task. As a consequence, the underline mechanism for the enhanced HER performance is still not well understood. It is well known that atomic layer deposition (ALD) can grow thin films with controllable thickness and composition on substrates with complex 3D structures layer by layer through alternating self-limiting surface reactions [Reference Zhao and Wang30, Reference Li, Guo and Wang31]. Compared to other methods to construct heterointerfaces, ALD can well-maintain the microstructure of the matrix, eliminating the effect of structural changes on catalytic performances. In addition, the composition and microstructure of the heterointerface can be controlled at the atomic level, which is of great importance to obtain a fundamental understanding about the contribution of heterointerfaces to electrocatalytic activities. There have been great progresses recently in developing ALD processes for various transition metal oxides [Reference Wang, Saadat, Xi, Lou, Molnar, Palacios and Gordon32, Reference Wang, Dong, Zhang, Liu, Ye and Gordon33, Reference Sheng, Wang, Wang, Wang and Xue34, Reference Su, Cui, Zhuo, Yang, Wang and Pan35, Reference Wang, Fu, Wang, Kong, Sheng, Wang, Chen and Xue36, Reference Gao, Shao, Yan, Li, Su, Meng and Wang37, Reference Lou, Zhou, Kim, Alghamdi, Gong, Feng, Wang, Ye and Gordon38, Reference Su, Xin, Feng, Lin, Wang, Liang, Zheng, Lin and Pan39, Reference Wang, Guo, Gao and Wang40, Reference Zhao, Gao, Guo, Su and Wang41, Reference Gao, Shi, Meng and Wang42] and sulfides [Reference Zhao and Wang30, Reference Li, Guo and Wang31, Reference Li, Gao, Shao, Su and Wang43, Reference Li, Shao, Su, Gao and Wang44, Reference Shao, Guo, Li, Su and Wang45, Reference Xiong, Guo, Li, Zhao and Wang46, Reference Guo and Wang47, Reference Wang, Guo, Xiong and Wang48, Reference Zhao, Guo and Wang49, Reference Wa, Xiong, Zhao, He, Chen and Wang50]. Xiong et al. [Reference Xiong, Guo, Li, Zhao and Wang46] designed and constructed a hybrid compound FexCo1−xSy electrocatalyst with controllable composition on a complex 3D structure (carbon nanotubes on carbon cloths) for HER by ALD. The best composition Fe0.54Co0.46S0.92 exhibited a remarkable HER performance with a fairly low overpotential of 70 mV to achieve 10 mA/cm2 current density in alkaline media. Li et al. [Reference Li, Guo and Wang31] deposited ultrathin Co9S8 on carbon nanotubes by ALD for the OER, oxygen reduction reaction (ORR), and rechargeable Zn–air batteries. The ALD-prepared Co9S8 on carbon nanotubes presented outstanding electrocatalytic performances both for the OER and ORR with high activity and stability. Wa et al. [Reference Wa, Xiong, Zhao, He, Chen and Wang50] developed a new strategy to prepare an efficient electrocatalyst for electrochemical glucose sensing. ALD was used to fabricate a pre-electrocatalyst of NiSx for Ni(OH)x with a rough surface and high electrocatalytic activity. All of these great efforts have demonstrated the capability of using the ALD technique for the rational design of high-performance energy materials.
In this work, we construct NiSx@MoS2 heterostructure electrocatalysts with controllable interface properties by growing NiSx on MoS2 nanosheets through ALD [Reference Meng, Wang, Geng, Ozgit-Akgun, Schneider and Elam51]. MoS2 nanosheets were synthesized by using a hydrothermal method on carbon cloths (CCs) with a large surface area. NiSx clusters were then deposited on the surface of MoS2 nanosheets by ALD to build the NiSx@MoS2 heterostructures. The amounts of NiSx loading on MoS2 surface were controlled by ALD cycle numbers, ranging from 5 to 100 cycles. Scanning electron microscopy (SEM), energy-dispersive X-ray spectroscopy (EDS), X-ray photoelectron spectroscopy (XPS), and X-ray diffraction (XRD) results confirmed the formation of NiSx@MoS2 heterostructures. Electrochemical measurement results showed that the NiSx@MoS2 heterostructures exhibit an enhanced HER performance with lower overpotential and faster dynamic process due to the synergetic effects between MoS2 and NiSx. The NiSx@MoS2 nanostructures with 25 cycles of NiSx possessed the optimal HER catalytic activity, with the overpotential of 165 mV at the current density of −10 mA/cm2, which is 74 mV lower than that of single-phase MoS2 nanosheets. Additionally, the NiSx@MoS2 heterostructure catalysts presented highly stability under operation. Our results can guild the design of high-performance electrocatalysts using ALD for other energy devices.
Results and discussion
First, the microstructure and HER activity of single-phase NiSx grown by ALD on CCs were evaluated. Figures 1(a) and 1(b) showed the SEM images of bare CCs surface and the CCs with NiSx grown by ALD with 100 cycles. The NiSx was found to be nanoclusters, which distributed homogeneously on the CCs surface [Fig. 1(b)]. To verify the formation of NiSx, EDS and XPS measurements were carried out to determine the composition of samples. As shown in Fig. 1(c), noticeable peaks of Ni and S can be observed in the EDS spectra. Figures 1(d) and 1(e) show the representative Ni 2p and S 2p XPS spectra of NiSx on CCs. The Ni 2p spectrum contains two sharp peaks located at 852.7 eV and 870.0 eV, which are attributed to the spin–orbit split of 2p 3/2 and 2p 1/2, respectively [Fig. 1(d)]. A doublet located at 162.0 eV and 163.0 eV was ascribed to the spin–orbit split of 2p 3/2 and 2p 1/2 in S, respectively. The S/Ni atomic ratio of NiSx was quantified to be ∼0.8, which is consistent with our previous work [Reference Li, Shao, Su, Gao and Wang44]. The HER catalytic activity of NiSx was examined by linear sweep voltammetry (LSV) measurement, which was performed in 1 M KOH aqueous solution using a standard three-electrode system with a slow sweep rate of 1 mV/s. Noting that all of the LSV polarization curves have been iR-corrected, where the series resistance R was determined by the current interrupt method. As shown in Fig. 1(f), the single-phase NiSx showed poor HER catalytic activity with an overpotential of 540 mV [versus reversible hydrogen electrode (RHE)] at the current density of −10 mA/cm2.

Figure 1: SEM images of (a) bare CCs, (b) NiSx on CCs deposited by ALD with 100 cycles. (c) EDS spectrum of the NiSx on CCs with 600 cycles (corresponding SEM image is shown in Fig. S1). (d) Ni 2p and (e) S 2p XPS spectra of NiSx on CCs. (f) LSV curves of bare CCs and NiSx grown by ALD with 100 cycles.
To construct NiSx@MoS2 heterostructures, MoS2 nanosheets were first synthesized on CCs by the hydrothermal method, and NiSx clusters were then deposited by ALD with different cycle numbers. The NiSx@MoS2 heterostructures with NiSx growth cycle numbers 5, 25, and 100 are denoted as 5NiSx@MoS2, 25NiSx@MoS2, and 100NiSx@MoS2, respectively.
The morphology of MoS2 nanosheets and NiSx@MoS2 heterostructures was probed by SEM, as shown in Fig. 2. MoS2 nanosheets synthesized by the hydrothermal method were found to cover the CCs surface uniformly, and showed large specific surface area and abundant edges [Fig. 2(a)]. The morphology of the inset image in Fig. 2(a) shows the surface of MoS2 nanosheets. As shown in Figs. 2(b) and 2(c), the nanosheet structure of the MoS2 matrix can be well maintained after NiSx deposition, while the surface of MoS2 nanosheets become rougher with the increase in cycle number. After 100 ALD cycles, NiSx in the form of nanoclusters were found to distribute uniformly on the surface of MoS2 nanosheets [Fig. 2(d) inset]. The NiSx clusters were formed because of the spontaneous agglomeration of Ni-containing species during ligand stripping in the H2S half-cycles, and these NiSx clusters became larger with the increase in cycle numbers [Reference Zhao and Wang30]. NiSx clusters anchored on the surface of MoS2 nanosheets to construct the NiSx@MoS2 heterostructures and offered abundant interfaces for the HER catalysis.

Figure 2: SEM images of (a) MoS2 nanosheets, (b) 5NiSx@MoS2, (c) 25NiSx@MoS2, and (d) 100NiSx@MoS2 heterostructures with the scale bar of 500 nm. The scale bars in the inset image of a and d are 100 nm.
The crystal structure of NiSx@MoS2 heterostructures was characterized by XRD and Raman measurements. As shown in Fig. 3, the XRD patterns of MoS2 nanosheets displayed diffraction peaks at 13.7°, 32.9°, 35.6°, and 57.7°, corresponding to the (002), (101), (103), and (110) planes of MoS2, respectively. One possible reason for the broad diffraction peaks and the absence of high-indexed diffraction peaks is the low crystallinity and short-range disordering nature of MoS2 grown by the hydrothermal method, which may offer a large amount of active sites for HER catalysis [Reference Xie, Zhang, Li, Grote, Zhang, Zhang, Wang, Lei, Pan and Xie52]. The small thickness of the MoS2 flake can also contribute to the broadening of the XRD peaks. The diffraction peak located at 25.9° was attributed to the carbon substrate. After ALD growth of NiSx, the XRD patterns showed negligible changes compared to the pristine ones, which indicates that the ALD deposition of NiSx did not change the microstructure of the MoS2 matrix (Fig. 3). This result suggests that ALD is an ideal approach for hetero-interface engineering. Due to the small volume of NiSx, no XRD peaks corresponding to NiSx were observed in the XRD patterns of NiSx@MoS2 heterostructures.

Figure 3: XRD patterns of MoS2 nanosheets, 5NiSx@MoS2, 25NiSx@MoS2, and 100NiSx@MoS2 heterostructures.
The Raman spectra of MoS2 nanosheets and NiSx@MoS2 heterostructures are shown in Fig. 4. Two Raman peaks at 405 cm−1 and 380 cm−1 can be observed, which were ascribed to the out-of-plane vibration of S atoms (A1′ peak) and the in-plane vibration of Mo and S atoms (E′ peak) in MoS2, respectively. Note that these two Raman peaks were with a large peak width, suggesting the high disorder and defective nature of MoS2 nanosheets, which is well consistent with the XRD results. It worth noting that no Raman peaks corresponding to NiSx were observed in the Raman spectra of NiSx@MoS2 heterostructures due to the small volume of NiSx.
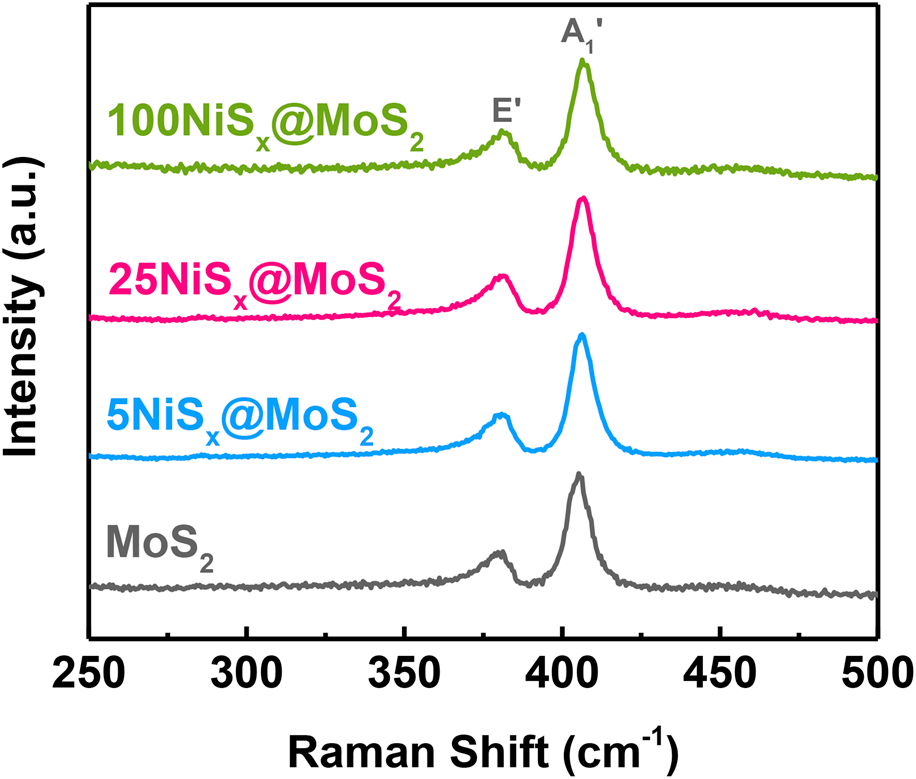
Figure 4: Raman spectra of MoS2 nanosheets, 5NiSx@MoS2, 25NiSx@MoS2, and 100NiSx@MoS2 heterostructures.
The HER activity of MoS2 nanosheets and NiSx@MoS2 heterostructures was systematically evaluated by electrochemical measurements. Figure 5(a) shows the LSV polarization curves for pristine MoS2 nanosheets and NiSx@MoS2 heterostructures with 5, 25, and 100 cycles of NiSx deposition. The pristine MoS2 nanosheets exhibit an overpotential of 239 mV (versus. RHE) at the current density of −10 mA/cm2. By constructing NiSx@MoS2 heterointerfaces, the HER performance had improved significantly as shown by the much lower overpotential in the LSV curves. The overpotentials at the current density of −10 mA/cm2 were 206, 165, and 181 mV for 5NiSx@MoS2, 25NiSx@MoS2, and 100NiSx@MoS2, respectively [Fig. 5(a)], which were 33, 74, and 58 mV lower than that for the pristine MoS2. The HER activity of NiSx@MoS2 heterostructures first increased with the ALD cycle number of NiSx and reached the maximum for 25NiSx@MoS2. However, as the cycle number further increased to 100, the HER performance decreased.

Figure 5: (a) LSV polarization curves, (b) electrochemical impedance spectroscopies, and (c) Tafel curves of MoS2 nanosheets, 5NiSx@MoS2, 25NiSx@MoS2, and 100NiSx@MoS2 heterostructures.
The electrochemical impedance spectroscopy (EIS) tests, which were performed at an overpotential of 150 mV, showed consistent results as the LSV measurement. As shown in Fig. 5(b), the EIS spectra exhibit a characteristic semicircle shape, which can be fitted by an ohmic resistance, a constant phase element, and a charge transfer resistance (R ct) [Fig. 5(b) inset]. The MoS2 single-phase and NiSx@MoS2 heterostructures show similar ohmic resistance values, which are from the solution and all ohmic contacts. But the charge transfer resistance (R ct), which is associated with the electrochemical reaction kinetics, is quite different between pristine MoS2 nanosheets and NiSx@MoS2 heterostructures. The R ct decreased significantly after introducing NiSx onto the MoS2 surface [Fig. 5(b)], which indicated a faster reaction kinetic of electrocatalysis for HER. The R ct was determined to be 38.22 ohm, 10.74 ohm, 4.52 ohm, and 6.61 ohm for the pristine MoS2 nanosheets, 5NiSx@MoS2, 25NiSx@MoS2, and 100NiSx@MoS2, respectively. The 25NiSx@MoS2 sample delivered the lowest R ct value (4.52 ohm), which is about one order of magnitude lower than the R ct value for pristine MoS2 (38.22 ohm), suggesting significantly improved HER activities. Moreover, the Tafel plots were found to be 147.4 mV/dec, 122.6 mV/dec, 104.2 mV/dec, and 119.4 mV/dec for pristine MoS2, 5NiSx@MoS2, 25NiSx@MoS2, and 100NiSx@MoS2 heterostructures, respectively. The 25NiSx@MoS2 possessed a Tafel slope (104.2 mV/decade) which was much smaller than that for MoS2 single-phase and NiSx@MoS2 heterostructures with other compositions [Fig. 5(c)]. According to the previous works [Reference Lin, Wang, Wang, Li, Si, Qi, Cao, Zhong, Fei and Feng3, Reference Ibupoto, Tahira, Tang, Liu, Morante, Fahlman, Arbiol, Vagin and Vomiero26], three principal steps are involved in the HER process in KOH aqueous solution:
(i) Volmer step, the formation of hydrogen adsorbed intermediates (Hads):

(ii) Heyrovsky step, the second electron transfer step:

(iii) Tafel step, the combination of Hads:

In general, it would be with a Tafel slope of 120, 40, or 30 mV/dec when the Volmer, Heyrovsky, or Tafel step acts as the rate-limiting and determining step for the HER, respectively [Reference Lin, Wang, Wang, Li, Si, Qi, Cao, Zhong, Fei and Feng3, Reference Ibupoto, Tahira, Tang, Liu, Morante, Fahlman, Arbiol, Vagin and Vomiero26]. In this work, the Tafel slope of the MoS2 single phase is 147.4 mV/dec, suggesting that the Volmer step is the rate-limiting step. After 25 cycles of NiSx deposited on the MoS2 surface, the 25NiSx@MoS2 heterostructure possess a Tafel slope of 104.2 mV/dec, which indicated that the rate-limiting step changes to the Volmer–Heyrovsky step, suggesting a facilitated electron transfer and improved adsorption for the reacting intermediates due to the formation of rich interfaces. It has been reported that the binding free energy of Hads intermediates is −0.33 eV for the pristine MoS2 catalyst sites, which is favorable to the hydrogen generation step (Heyrovsky step or Tafel step). However, the MoS2 exhibits a high barrier of 1.17 eV for H–OH adsorption, which hinders the dissociation of H2O to form Hads intermediates (Volmer step), resulting in the sluggish hydrogen evolution kinetics [Reference Zhang, Liu, Wang, Ruan, Ji, Xu, Chen, Wan, Miao and Jiang53]. Zhang et al. [Reference Zhang, Wang, Pohl, Rellinghaus, Dong, Liu, Zhuang and Feng29] suggested that the presence of NiSx@MoS2 interfaces could facilitate the chemisorption of hydrogen and oxygen-containing intermediates, which can promote the initial water dissociation step (Volmer step). Furthermore, density functional theory (DFT) calculation [Reference Zhang, Wang, Pohl, Rellinghaus, Dong, Liu, Zhuang and Feng29] also showed that the chemisorption free energies of hydrogen and hydroxide on the interfaces were lower than that on the surface of MoS2 and NiSx single phases. As a result, the interfaces between MoS2 and NiSx show a synergistic effect that MoS2 exhibits a favorable free energy for Hads intermediates and NiSx can promote the adsorption of oxygen-containing intermediates, which may accelerate the HER processes, resulting in the enhanced electrocatalytic performance.
All the electrochemical measurement results consistently suggest that the HER catalytic activity of NiSx@MoS2 heterostructures is significantly higher than that of MoS2 single-phase nanosheets. Since pure NiSx grown by ALD showed negligible contribution to the HER activity [Figs. 1(d) and 5(a)], the enhanced HER activity of NiSx@MoS2 was likely due to the presence of NiSx/MoS2 heterointerfaces. As shown in the electrochemical test results and in previous literatures [Reference Lin, Wang, Wang, Li, Si, Qi, Cao, Zhong, Fei and Feng3, Reference Zhang, Wang, Pohl, Rellinghaus, Dong, Liu, Zhuang and Feng29], the presence of NiSx/MoS2 heterointerfaces can provide rich electrocatalytic active sites, enhancing the adsorption of reaction intermediates, improving electronic conductivity, and facilitating the electron transfer process. It is important to note that the HER performance of NiSx@MoS2 heterostructures first improved with the NiSx cycle number, but reached the maximum for the cycle number of 25. As discussed above, the area near the NiSx, MoS2, and electrolyte triple-phase boundary is believed to be the activity region of the HER. As the ALD cycle number increased, such active regions first increased because of more NiSx and MoS2 interfacial regions being exposed to the electrolytes, leading to improved activity. Further increase in NiSx coverage resulted in the decrease of the triple-phase boundary region, which led to the degradation of HER activity as observed above for 100NiSx@MoS2.
Operational stability is another critical factor that should be taken into account in practical applications. Thereby, the long-term stability of NiSx@MoS2 heterostructures was also evaluated. The chronopotentiometry measurement (CP) was performed at the current density of −10 mA/cm2 for 20 h in 1 M KOH. The overpotential at the current density of −10 mA/cm2 of MoS2 and 25NiSx@MoS2 samples was compared over time. As shown in Fig. 6, both MoS2 and 25NiSx@MoS2 remain stable during operation, indicating a firm combination of MoS2 and NiSx and a negligible exfoliation of NiSx clusters over operation. The overpotential of 25NiSx@MoS2 is still 60 mV lower than that of pristine MoS2, suggesting good long-term stability and excellent HER performance.

Figure 6: Chronopotentiometry curves at the current density of −10 mA/cm2 of MoS2 nanosheets and 25NiSx@MoS2 heterostructure.
Conclusion
In summary, we developed a novel strategy to construct NiSx@MoS2 heterostructures controllably through the combination of a hydrothermal method and ALD techniques. MoS2 nanosheets with a large surface area and abundant edge sites were synthesized on CCs substrates. After ALD deposition, homogeneous NiSx clusters were anchored on the surface of MoS2 nanosheets. SEM and XRD results confirmed that the morphology and structure of MoS2 can be well maintained during the ALD process. NiSx@MoS2 heterostructures exhibited a strongly enhanced HER performance with lower overpotential and faster dynamic process compared with single-phase MoS2 nanosheets. The NiSx@MoS2 heterostructures with 25 ALD cycles of NiSx deposition showed the optimal HER catalytic activity, with a decreased overpotential of 74 mV at the current density of −10 mA/cm2 than the pristine MoS2 nanosheets. Additionally, the NiSx@MoS2 heterostructure catalysts present highly stability under operation in alkaline media. The high electrocatalytic activity of NiSx@MoS2 for HER was attributed to the presence of NiSx/MoS2 heterointerfaces, which not only provide high-density electrocatalytic active sites for the adsorption of reaction intermediates but also promote the electron transfer process. While NiSx@MoS2 was taken as the model system in this work, the methodology and knowledge can also apply to construct other transition metal oxide or sulfide heterostructure electrocatalysts for various reactions, such as OER, ORR, and CO2 reduction reaction.
Experimental section
Synthesis of MoS2 nanosheets and NiSx@MoS2 heterostructures
MoS2 nanosheets were synthesized by a hydrothermal method. Ammonium molybdate tetrahydrate [(NH4)6Mo7O24·4H2O] and thiourea (CH4N2S) were dissolved in water and sonicated for 30 min to form a homogeneous and pellucid solution, which was then transferred into a Teflon-lined stainless-steel autoclave for MoS2 synthesis. The pH of the solution was adjusted to 3. The autoclave was heated to 180 °C for 24 h and then allowed to cool to room temperature naturally. CCs was used as the substrate for growing the MoS2 nanosheets. The resulting samples were washed with ultra-pure water several times to remove the excess reactant and then dried at 60 °C overnight. The NiSx was grown on MoS2 nanosheets by ALD at 120 °C using bis(N,N′-di-tert-butylacetamidinato)nickel(II) (Ni(amd)2) and H2S as precursors in a home-built tubular reactor. Details of the NiSx ALD process are reported in our previous works [Reference Zhao and Wang30, Reference Li, Shao, Su, Gao and Wang44, Reference Zhao, Guo and Wang49]. The amount of NiSx deposited on MoS2 was controlled by the number of ALD cycles, ranging from 5 to 100 cycles.
Characterization
The morphologies of NiSx@MoS2 heterostructures, and NiSx and MoS2 single-phase reference samples were characterized by high-resolution field emission SEM (SU8010, Hitachi, Tokyo, Japan). Electron energy of 5 kV was used for morphology characterization. EDS was carried out to determine the chemical composition of the materials. The crystal structures of the materials were characterized by XRD using Bruker D8 Advance (Karlsruhe, Germany) with monochromatized Cu Kα radiation. The Raman spectra were measured by a confocal microscopic system (LabRAM HR Evolution, Horiba, Pairs, France) equipped with a green laser (λ = 532 nm, Laser Quantum Ltd., Stockport, U.K.), and a 100× objective lens was used to focus the laser to a spot with size of 1 μm.
Electrochemical measurements
The HER performance of samples was measured by a CHI 660E electrochemical workstation (CH Instruments, Shanghai, China) using a standard three-electrode system in 1 M KOH aqueous solution. The NiSx@MoS2 heterostructures, and MoS2 and NiSx single-phase reference samples on CCs served as the working electrode, and a carbon rod was used as the counter electrode. A Ag/AgCl electrode prefilled with saturated KCl aqueous solution was used as the reference electrode. LSV measurements were carried out at 1 mV/s. EIS was measured at 150 mV versus RHE with an amplitude of 5 mV and a frequency range from 106 to 10−2 Hz. Chronopotentiometry tests were carried out at a constant current density of −10 mA/cm2 for 20 h.
Supplementary material
To view supplementary material for this article, please visit https://doi.org/10.1557/jmr.2019.325.
Acknowledgment
This work was supported by the National Natural Science Foundation of China (11605063, 51672011, and 11975102), Guangzhou Science and Technology Program General Projects (201707010146), the Fundamental Research Funds for the Central Universities (2018MS40), State Key Laboratory of Pulp and Paper Engineering (2018TS08), Guangdong Pearl River Talent Program (2017GC010281), and Guangdong Innovative and Entrepreneurial Research Team Program (2014ZT05N200) and Natural Science Foundation of Fujian Province (No. 2015J01068).