Introduction
F-type adenosine triphosphate (ATP) synthases (also called F-ATPases of F1F0-ATPases) are bidirectional turbine-like enzymes coupling ATP synthesis or hydrolysis with proton translocation through biological membranes in bacteria and their endosymbiotic descendants, mitochondria and chloroplasts (for recent reviews see Junge and Nelson, Reference Junge and Nelson2015; Walker, Reference Walker and Wikstrom2017; Kuhlbrandt, Reference Kuhlbrandt2019). When operating in the forward mode, ATP synthases utilize the proton motive force to produce ATP and thus represent fundamental constituents of the oxidative phosphorylation pathway. In the reverse mode, they act as ATP-consuming proton pumps contributing to the generation of electrochemical membrane potential. Bacteria employ both modes depending on the species and growth conditions (Cotter and Hill, Reference Cotter and Hill2003). By contrast, mitochondrial ATP synthases in most aerobic eukaryotes function as a major source of cellular ATP and the reversal of their activity is a manifestation of pathophysiological conditions (Campanella et al., Reference Campanella, Parker, Tan, Hall and Duchen2009). Unlike the monomeric bacterial and chloroplastic counterparts, all mitochondrial ATP synthases characterized so far occur in dimers (Arnold et al., Reference Arnold, Pfeiffer, Neupert, Stuart and Schagger1998; Dudkina et al., Reference Dudkina, Heinemeyer, Keegstra, Boekema and Braun2005). The wide phylogenetic distribution of the ATP synthase dimers suggests that the enzyme's dimerization is a common feature of all aerobic eukaryotes with oxidative phosphorylation and was possibly present already in the last eukaryotic common ancestor. Dimerization of ATP synthases induces curvature of the inner mitochondrial membrane (Dudkina et al., Reference Dudkina, Sunderhaus, Braun and Boekema2006; Davies et al., Reference Davies, Anselmi, Wittig, Faraldo-Gomez and Kuhlbrandt2012) and the intrinsic propensity of dimers to self-assemble into rows facilitates membrane shaping and governs cristae formation (Anselmi et al., Reference Anselmi, Davies and Faraldo-Gomez2018; Blum et al., Reference Blum, Hahn, Meier, Davies and Kühlbrandt2019). Spatial separation of ATP synthases and electron transport chain (ETC) complexes on rims and flat regions of cristae, respectively, creates a local proton concentration gradient in cristae lumen, enhancing oxidative phosphorylation (Davies et al., Reference Davies, Strauss, Daum, Kief, Osiewacz, Rycovska, Zickermann and Kuhlbrandt2011). Therefore, apart from the enzymatic function, ATP synthases affect mitochondrial physiology by aiding in the determination of submitochondrial ultrastructure.
In Trypanosoma brucei, a medically relevant digenetic parasite, the mitochondrial ATP synthase exhibits a 2-fold deviation from its counterparts from traditional model organisms. First, its architecture differs markedly from canonical ATP synthases. Second, its role switches during the parasite's life cycle, from being an ATP producer in the insect form to an ATP consumer maintaining the vital mitochondrial membrane potential in the mammalian bloodstream form (BSF). This switch is associated with major rearrangement of mitochondrial morphology, possibly involving altered ATP synthase oligomerization. Unlike in T. brucei, in Trypanosoma cruzi and Leishmania, ATP synthase acts true to its name and participates in the oxidative phosphorylation providing ATP in all life forms of the parasite. In the first part of this review, we discuss the recent progress in our understanding of ATP synthase architecture based on structural characterization of enzymes from various eukaryotic supergroups with a focus on trypanosomal ATP synthase complex. In the second part, we summarize our knowledge of the ATP synthase importance for the disease-causing forms of medically relevant trypanosomatid parasites.
Unexpected diversity of mitochondrial ATP synthases
Structural studies of mitochondrial ATP synthases have been key for the understanding of molecular mechanisms of ATP synthesis. The seminal research on the structure of the matrix-facing soluble F1-ATPase subcomplex isolated from the bovine heart (Abrahams et al., Reference Abrahams, Leslie, Lutter and Walker1994) initiated two decades of dominance of X-ray crystallography in the field. Numerous crystal structures of subcomplexes of bovine and yeast ATP synthases provided atomic models of the enzyme's rotor and the extrinsic part of the peripheral stalk, and revealed the mechanism of the conversion of the rotational force into the cyclic catalysis of ATP formation (reviewed in Junge and Nelson, Reference Junge and Nelson2015; Walker, Reference Walker and Wikstrom2017). Due to the striking similarity of the blueprints for bacterial and opisthokontal mitochondrial F-type ATP synthases and their fundamental role in cellular energetics, it was assumed that the mitochondrial enzymes do not differ substantially between eukaryotic lineages. However, only the advance of cryo-electron microscopy (cryo-EM) techniques in the last few years allowed researchers to build atomic models of complete mitochondrial ATP synthases not only from conventional model organisms [mammals (Gu et al., Reference Gu, Zhang, Zong, Guo, Liu, Yi, Wang, Zhuo and Yang2019; Pinke et al., Reference Pinke, Zhou and Sazanov2020; Spikes et al., Reference Spikes, Montgomery and Walker2020) and Saccharomyces cerevisiae (Guo et al., Reference Guo, Bueler and Rubinstein2017)], but also from protists representing other eukaryotic phyla [Polytomella (Murphy et al., Reference Murphy, Klusch, Langer, Mills, Yildiz and Kuhlbrandt2019), Euglena (Muhleip et al., Reference Muhleip, McComas and Amunts2019), Tetrahymena (Flygaard et al., Reference Flygaard, Mühleip, Tobiasson and Amunts2020) and Toxoplasma (Mühleip et al., Reference Mühleip, Kock Flygaard, Ovciarikova, Lacombe, Fernandes, Sheiner and Amunts2021)]. From the mechanistic point of view, structures revealed by cryo-EM elucidated how the proton motive force across biological membranes is converted into the torque of the enzyme's rotor (Klusch et al., Reference Klusch, Murphy, Mills, Yildiz and Kuhlbrandt2017; Hahn et al., Reference Hahn, Vonck, Mills, Meier and Kuhlbrandt2018) and how the symmetry mismatch of the rotor and catalytic subcomplexes is compensated by the flexibility of the peripheral stalk (Sobti et al., Reference Sobti, Ishmukhametov, Bouwer, Ayer, Suarna, Smith, Christie, Stocker, Duncan and Stewart2019). From the evolutionary perspective, the structures demonstrated that although the functional core is universally conserved in all F-type ATP synthases, peripheral parts and the dimerization interface of mitochondrial ATP synthases have diverged substantially among eukaryotic lineages. Notably, cryo-EM structures not only allowed identification of novel lineage-specific components, but in some species also revealed conserved subunits, which had not been previously identified by traditional homology searches using known genomes and proteomes (Fig. 1).

Fig. 1. Structural diversity of mitochondrial ATP synthases mapped on the phylogenetic tree of eukaryotes. The figure summarizes structural and proteomic studies of mitochondrial ATP synthases. The phylogenetic tree is based on Burki et al. (Reference Burki, Roger, Brown and Simpson2020). Organisms with ATP synthases with atomic models obtained by single particle cryo-EM, visualized by cryo-ET (see first two columns) or characterized by MS, and several relative species were included. Major groups without experimental data are shown in small font. Numbers of subunits showed in grey are based on proteomic characterization of purified complexes without available single particle cryo-EM analysis and might be revised in future. ‘Canonical’ subunits are proteins originally identified in opisthokonts, which have divergent homologs in other lineages. Categorization of ATP synthases in types I to IV is based on Kuhlbrandt (Reference Kuhlbrandt2019). Cristae morphology is adopted from Panek et al. (Reference Panek, Elias, Vancova, Lukes and Hashimi2020). The numbered references are following: 1 (Muhleip et al., Reference Muhleip, Dewar, Schnaufer, Kuhlbrandt and Davies2017), 2 (Zikova et al., Reference Zikova, Schnaufer, Dalley, Panigrahi and Stuart2009), 3 (Muhleip et al., Reference Muhleip, McComas and Amunts2019), 4 (Sathish Yadav et al., Reference Sathish Yadav, Miranda-Astudillo, Colina-Tenorio, Bouillenne, Degand, Morsomme, Gonzalez-Halphen, Boekema and Cardol2017), 5 (Davies et al., Reference Davies, Anselmi, Wittig, Faraldo-Gomez and Kuhlbrandt2012), 6 (Guo et al., Reference Guo, Bueler and Rubinstein2017), 7 (Davies et al., Reference Davies, Strauss, Daum, Kief, Osiewacz, Rycovska, Zickermann and Kuhlbrandt2011), 8 (Spikes et al., Reference Spikes, Montgomery and Walker2020), 9 (Mühleip et al., Reference Mühleip, Kock Flygaard, Ovciarikova, Lacombe, Fernandes, Sheiner and Amunts2021), 10 (Salunke et al., Reference Salunke, Mourier, Banerjee, Pain and Shanmugam2018), 11 (Huet et al., Reference Huet, Rajendran, van Dooren and Lourido2018), 12 (Flygaard et al., Reference Flygaard, Mühleip, Tobiasson and Amunts2020), 13 (Muhleip et al., Reference Muhleip, Joos, Wigge, Frangakis, Kuhlbrandt and Davies2016), 14 (Blum et al., Reference Blum, Hahn, Meier, Davies and Kühlbrandt2019), 15 (Murphy et al., Reference Murphy, Klusch, Langer, Mills, Yildiz and Kuhlbrandt2019), 16 (Vazquez-Acevedo et al., Reference Vazquez-Acevedo, Cardol, Cano-Estrada, Lapaille, Remacle and Gonzalez-Halphen2006), 17 (Klodmann et al., Reference Klodmann, Senkler, Rode and Braun2011), 18 (Bultema et al., Reference Bultema, Braun, Boekema and Kouril2009), 19 (Senkler et al., Reference Senkler, Senkler, Eubel, Hildebrandt, Lengwenus, Schertl, Schwarzlander, Wagner, Wittig and Braun2017).
All F-type ATP synthases are composed of two subcomplexes, the membrane-embedded F0 and the soluble F1, connected by a rotary central stalk and a stationary peripheral stalk (Fig. 2). The globular F1 subcomplex, also referred to as F1-ATPase, is a pseudo-symmetrical assembly of three heterodimers of α- and β-subunits organized around single γ-subunit, the main constituent of the central stalk. The functional core of F0 consists of the a-subunit and a ring of several identical c-subunits (c-ring), providing together a path for protons across the membrane. Proton translocation drives the rotation of the c-ring and the tightly attached central stalk. The torque of the asymmetric γ-subunit induces cyclic conformational changes of three active sites on the interfaces of α- and β-subunits, resulting in the stepwise binding of ADP and phosphate, their condensation into ATP, and its release, emptying the nucleotide binding site for the next catalytic cycle (Abrahams et al., Reference Abrahams, Leslie, Lutter and Walker1994). The (αβ)3-hexamer is prevented from rotation by interaction with the oligomycin sensitivity-conferring protein (OSCP), the uppermost constituent of the extrinsic part of the peripheral stalk.
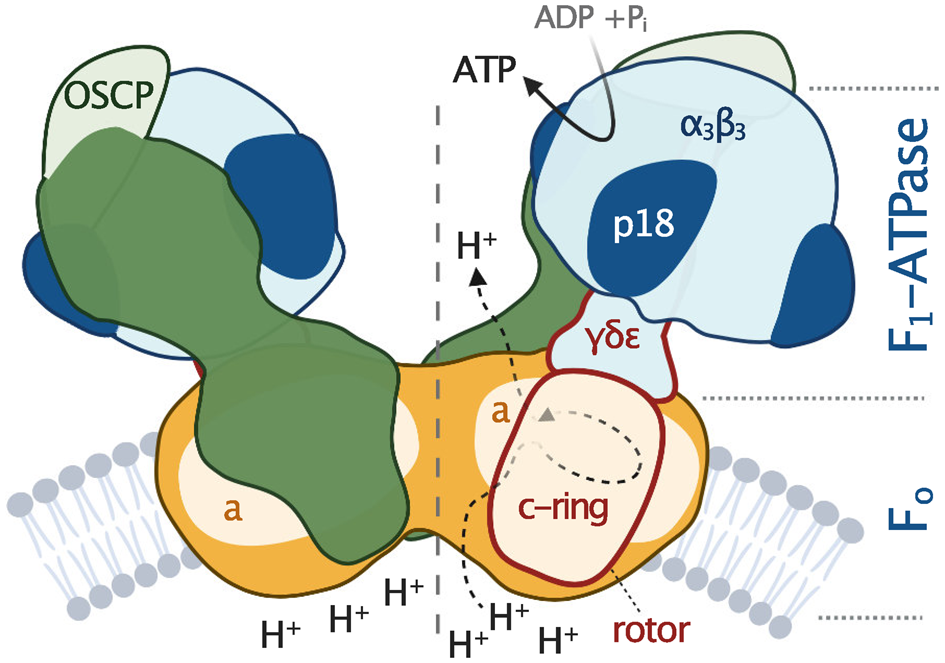
Fig. 2. Schematic depiction of euglenozoan mitochondrial ATP synthase in the ATP production mode. The F1-ATPase, peripheral stalk and membrane-embedded part are shown in shades of blue, green and orange, respectively. Subunits identified in all reported mitochondrial ATP synthases are pale. The dark green and dark orange regions are composed of conserved and lineage-specific subunits. The p18 subunit is restricted to Euglenozoa. The rotor is outlined in red.
The entire F1-ATPase consisting of subunits α 3, β 3, γ, δ and ɛ (stoichiometry of subunits indicated by the subscripts), OSCP and both proton translocating components (a-subunit and c-ring) are present in all reported mitochondrial ATP synthases. The rest of the enzyme is markedly less conserved (Fig. 2). The highest diversity is observed in the soluble part of the peripheral stalk and in the lumenal and peripheral F0 regions, often composed of lineage-specific subunits or lineage-specific extensions of conserved proteins. Subunits involved in the anchoring of the peripheral stalk to the membrane and in clamping the a-subunit to the c-ring (b, d, e, f, g, i/j, k and 8), originally identified in opisthokonts, appear to be conserved in several other eukaryotic groups (Fig. 1). In many cases, their proposed homology is based largely on tertiary and quaternary structure resemblances revealed by cryo-EM. Low sequence similarity precludes identification of the respective homologs in lineages without structurally characterized ATP synthases. Although dimerization is a general hallmark of all reported mitochondrial ATP synthases, subunits constituting the dimer interface differ between eukaryotic lineages, likely due to divergent evolution. Consequently, the shape of dimers and topography of oligomeric assemblies vary in the characterized enzymes, which is reflected in diverse cristae morphology (Fig. 1; Dudkina et al., Reference Dudkina, Oostergetel, Lewejohann, Braun and Boekema2010; Davies et al., Reference Davies, Anselmi, Wittig, Faraldo-Gomez and Kuhlbrandt2012; Muhleip et al., Reference Muhleip, Joos, Wigge, Frangakis, Kuhlbrandt and Davies2016; Muhleip et al., Reference Muhleip, Dewar, Schnaufer, Kuhlbrandt and Davies2017; Mühleip et al., Reference Mühleip, Kock Flygaard, Ovciarikova, Lacombe, Fernandes, Sheiner and Amunts2021).
Unique structural features of ATP synthases in Trypanosomatida
The knowledge about the architecture of mitochondrial ATP synthases in Trypanosomatida, a group of protozoan parasites with monoxenous or dixenous life cycles, is based mostly on studies performed with cultured insect trypomastigotes of T. brucei. The composition of the mitochondrial ATP synthase in these cells was determined by mass spectrometry (MS) identification of proteins co-immunoprecipitated with F1 and tandem affinity purified using TAP-tagged conserved or newly identified subunits as baits. These biochemical analyses complemented by a comprehensive genome search revealed 23 subunits (Zikova et al., Reference Zikova, Schnaufer, Dalley, Panigrahi and Stuart2009), including the a-subunit detected by MS later (Skodova-Sverakova et al., Reference Skodova-Sverakova, Horvath and Maslov2015). Characterization of the trypanosomal F1-ATPase released by chloroform from mitochondrial membrane fragments and purified by two-step chromatography (Gahura and Zikova, Reference Gahura and Zikova2019) showed that the catalytic subcomplex contains three copies of the euglenozoan-specific p18-subunit in addition to the universally conserved subunits α, β, γ, δ and ɛ (Gahura et al., Reference Gahura, Subrtova, Vachova, Panicucci, Fearnley, Harbour, Walker and Zikova2018b). The F1-ATPase structure determined by X-ray crystallography further revealed that each copy of p18-subunit, an α-helical protein with three pentatricopeptide repeats, associates peripherally with one of the three α chains (Fig. 3A), and does not contribute directly to the catalytic mechanism (Montgomery et al., Reference Montgomery, Gahura, Leslie, Zikova and Walker2018). Although the atomic structure did not explain the function of p18, the elaboration of the F1 head in Euglenozoa by this additional subunit is extraordinary, because the α 3β 3γ subcomplex is invariant in all other known F-type ATP synthases. In addition, the α-subunit was found to be proteolytically cleaved at two sites separated by eight amino acid residues, producing two fragments α 1–127 and α 135–560, both stably associated with the complex. The split of α-subunit into two parts was also demonstrated in other euglenozoan protists Crithidia fasciculata (Speijer et al., Reference Speijer, Breek, Muijsers, Hartog, Berden, Albracht, Samyn, Van Beeumen and Benne1997), Leishmania tarentolae (Nelson et al., Reference Nelson, Aphasizheva, Falick, Nebohacova and Simpson2004) and Euglena gracilis (Sathish Yadav et al., Reference Sathish Yadav, Miranda-Astudillo, Colina-Tenorio, Bouillenne, Degand, Morsomme, Gonzalez-Halphen, Boekema and Cardol2017). The structure of the trypanosomal F1-ATPase showed that the cleavage occurs at the region corresponding to a loop on the surface of F1-ATPases from other organisms and splits the N-terminal β-barrel domain from the rest of the protein (Montgomery et al., Reference Montgomery, Gahura, Leslie, Zikova and Walker2018; Fig. 3A and B). Nevertheless, the architecture of the entire (αβ)3-headpiece, including the nucleotide binding pockets, is highly similar to the prototypical bovine F1-ATPase (Bowler et al., Reference Bowler, Montgomery, Leslie and Walker2007) and the biological relevance of the α-subunit cleavage remains unclear.

Fig. 3. Structural traits of euglenozoan mitochondrial ATP synthases. (A) Structure of F1-ATPase from Trypanosoma brucei determined by X-ray crystallography (Montgomery et al., Reference Montgomery, Gahura, Leslie, Zikova and Walker2018). (B) The α-subunit in euglenozoans is split by proteolytic cleavage at two sites. The euglenozoan-specific subunit p18 associates with the C-terminal fragment α 135–560 and does not contact any other F1 component. (C) Structure of the ATP synthase dimer from Euglena gracilis (Muhleip et al., Reference Muhleip, McComas and Amunts2019). Elements with and without homology in T. brucei are shown in surface and white cartoon representation, respectively. F1-ATPase and c-ring are in pale blue. Subunits with newly proposed homology between Euglena and Trypanosoma are coloured individually and labelled with names used in both species (Perez et al., Reference Perez, Lapaille, Degand, Cilibrasi, Villavicencio-Queijeiro, Morsomme, Gonzalez-Halphen, Field, Remacle, Baurain and Cardol2014; Muhleip et al., Reference Muhleip, McComas and Amunts2019). The sequence identities of respective homolog pairs are shown. All other peripheral stalk and F0 subunits are in dark blue. (D) Interaction of the peripheral stalk with F1-ATPase. The canonical interaction interface and lineage-specific contacts between OSCP and p18 are shown. (E) Superposition of the a-subunit and adjacent conserved transmembrane helices from E. gracilis (subunits coloured individually) and bovine [a-subunit white, all other subunits in grey; PDB ID 6ZPO (Spikes et al., Reference Spikes, Montgomery and Walker2020)] ATP synthase. (F) Dimer interface of the ATP synthase in E. gracilis. Subunits involved in inter-monomer contacts are shown in cartoon representation. Regions that are present in T. brucei counterparts based on homology modelling with Swiss model (Waterhouse et al., Reference Waterhouse, Bertoni, Bienert, Studer, Tauriello, Gumienny, Heer, de Beer, Rempfer, Bordoli, Lepore and Schwede2018) are coloured individually and Euglena-specific elements are in white. All other subunits are shown as transparent surfaces. Ordered lipids (cardiolipins and phosphatidylcholines or phosphatidylethanolamines) localizing to the dimer interface are shown as sticks.
So far, there is no available structure of a complete ATP synthase from any trypanosomatid species. However, low-resolution structures obtained by subtomogram averaging documented general morphological similarity between ATP synthase dimers from T. brucei and E. gracilis, a representative of euglenids, a lineage closely related to kinetoplastids (Muhleip et al., Reference Muhleip, Dewar, Schnaufer, Kuhlbrandt and Davies2017). An atomic model of dimeric ATP synthase from E. gracilis has recently been determined by cryo-EM (Muhleip et al., Reference Muhleip, McComas and Amunts2019). Apart from the universally conserved components of mitochondrial ATP synthases (α, β, γ, δ, ɛ, a, c and OSCP), the E. gracilis enzyme contains seven subunits with previously recognized homology (Sathish Yadav et al., Reference Sathish Yadav, Miranda-Astudillo, Colina-Tenorio, Bouillenne, Degand, Morsomme, Gonzalez-Halphen, Boekema and Cardol2017) to proteins found in the complex from T. brucei (Zikova et al., Reference Zikova, Schnaufer, Dalley, Panigrahi and Stuart2009). To get a deeper insight into the trypanosomal ATP synthase structure, we performed systematic pairwise sequence comparison of all remaining subunits in the complex from E. gracilis with all non-conserved trypanosomal ATP synthase components, and propose homology of four additional protein pairs, which exhibit >20% sequence identity (Fig. 3C). Overall, 19 of 23 subunits of the trypanosomal ATP synthase have homologs found in the structure from E. gracilis. Taken together, although the ATP synthases from E. gracilis and T. brucei are not compositionally identical, it is conceivable to assume that the structural features shaped by the conserved subunits are shared between the two species (Fig. 3C), and possibly among Euglenozoa in general.
The peripheral stalk in euglenozoan ATP synthases differs markedly from other lineages. The universally conserved OSCP features a long and partially flexible C-terminal extension, which bridges the core of the protein on the top of F1 with ATPTB2, a highly divergent and extended homolog of the d-subunit from opisthokontal ATP synthases anchoring the peripheral stalk to the membrane. The interaction of ATPTB2 with the extension of OSCP is clamped between two laterally positioned lineage-specific globular proteins ATPTB3 and ATPTB4 on one side and one copy of p18 on the other side. The contacts of the peripheral stalk with p18 contribute to the immobilization of the (αβ)3-hexamer (Fig. 3D). However, the reinforcement of the interaction between the F1 head and the peripheral stalk is unlikely the only role of p18, because the protein is essential for the integrity of the F1-ATPase (Gahura et al., Reference Gahura, Subrtova, Vachova, Panicucci, Fearnley, Harbour, Walker and Zikova2018b), in contrast to the peripheral stalk components OSCP (Hierro-Yap et al., Reference Hierro-Yap, Subrtová, Gahura, Panicucci, Dewar, Chinopoulos, Schnaufer and Zikova2021) and ATPTB2/d-subunit (Subrtova et al., Reference Subrtova, Panicucci and Zikova2015). In E. gracilis, the extensions of OSCP and d-subunit compensate for the reduction of the b-subunit, the major constituent of the peripheral stalk in bacteria and mitochondria of mammals and yeast. Sequence comparison did not reveal a homolog of the b-subunit in the ATP synthase of T. brucei, suggesting that it might be further reduced or completely absent in Trypanosomatida.
In all reported F-type ATP synthases, the proton channel-forming a-subunit contains two horizontal membrane-embedded helices H5 and H6, which are directly involved in proton translocation (Guo et al., Reference Guo, Bueler and Rubinstein2017; Murphy et al., Reference Murphy, Klusch, Langer, Mills, Yildiz and Kuhlbrandt2019). In mammals (Spikes et al., Reference Spikes, Montgomery and Walker2020) and yeast (Guo et al., Reference Guo, Bueler and Rubinstein2017) the two helices are lined by a series of six parallel transmembrane helices, contributed by one helix of each of subunits b, f, 8, i/j and k, and by a transmembrane helix of the a-subunit itself. The same configuration is observed in E. gracilis (Fig. 3E), suggesting the conservancy of the contributing subunits. Although the ATP synthase in T. brucei contains homologs of E. gracilis subunits k and i/j, the homologs of subunits b, f and 8 were not identified. The dimer interface of the ATP synthase in E. gracilis is formed largely by subunits ATPEG1 and ATPEG2 without discernible homology outside Euglenozoa, but with apparent homologs in Trypanosomatida (called ATPTB9 and ATPTB8 in T. brucei; Perez et al., Reference Perez, Lapaille, Degand, Cilibrasi, Villavicencio-Queijeiro, Morsomme, Gonzalez-Halphen, Field, Remacle, Baurain and Cardol2014; Fig. 3C and F). The presence of these two proteins in T. brucei together with the fact that the dimers from both species arrange into short left-handed helices, as showed by cryo-electron tomography (cryo-ET; Muhleip et al., Reference Muhleip, Dewar, Schnaufer, Kuhlbrandt and Davies2017), strongly suggests that the dimerization is mediated by the same elements. The dimer in E. gracilis is further stabilized by a homotypic interaction of extensions of d-subunit adopting a ferredoxin-like fold on the matrix side of the membrane. This extension is specific to euglenids and the interaction is therefore completely missing in T. brucei, possibly explaining the poor stability of its ATP synthase dimers (our observation). Notably, the dimer interface in Euglena contains several ordered phospholipid molecules, predominantly cardiolipins (Muhleip et al., Reference Muhleip, McComas and Amunts2019; Fig. 3F). Molecules of cardiolipin are presumably integrated also in the trypanosomal ATP synthase, as cardiolipin plays a vital role in the stability of the complex (Serricchio et al., Reference Serricchio, Hierro-Yap, Schadeli, Ben Hamidane, Hemphill, Graumann, Zikova and Butikofer2020).
Role of the ATP synthase in trypanosomatid parasites
The function of the ATP synthase in trypanosomatid parasites depends on the species and their life cycle stage. Digenetic trypanosomatid parasites (e.g. T. brucei, T. cruzi and Leishmania) possess a complex life cycle as they alternate between the insect vector and a mammalian host. In humans, they cause dreadful diseases (African trypanosomiasis, Chagas disease and leishmaniases). The scarcity of effective treatments and weak vaccine prospects drive an ongoing urgent need to identify new therapeutic targets. Considering the ATP synthase's central position in energy metabolism and its structural and compositional divergence from the mammalian counterpart, one may consider this complex as a promising drug target. The feasibility of targeting the ATP synthase is underscored by the recent approval of bedaquiline, a specific F-ATP synthase inhibitor that blocks the c-ring rotation, to treat multi-drug resistant tuberculosis (Preiss et al., Reference Preiss, Langer, Yildiz, Eckhardt-Strelau, Guillemont, Koul and Meier2015). Whereas the function of the ATP synthase in T. brucei parasites is well understood, the exact role of the ATP synthase and its requirement for virulence and survival of Leishmania and T. cruzi parasites are not well defined yet.
Role of the ATP synthase in intracellular amastigotes of Leishmania and T. cruzi parasites
Leishmania parasites infect a mammalian host when an infected sand fly takes a blood meal. The promastigote form is released to the skin and is rapidly internalized by macrophages. Inside the macrophages, promastigotes differentiate to aflagellated and non-motile amastigotes, which multiply in the acidic environment of the phagolysosome-like parasitophorous vacuole, whose exact metabolic content has not been determined yet. The environment most likely offers a wide range of carbon sources (sugars, amino acids and fatty acids) for the parasite to salvage due to macrophage polarization to the M2 state, which exhibits increased oxidative metabolism and promotes Leishmania parasites growth (Saunders and McConville, Reference Saunders and McConville2020). Despite this seemingly plentiful environment, intracellular amastigotes do not proliferate rapidly (Kloehn et al., Reference Kloehn, Saunders, O'Callaghan, Dagley and McConville2015), but rather activate a stringent metabolic response, a protective mechanism to confer resistance to multiple cellular stresses (oxidative, nutritional and pH). This programmed response is associated with reduced rates of glucose uptake and with low metabolic activity to possibly minimize reactive oxygen species (ROS)-sensitive processes and to lessen the levels of endogenously generated ROS (McConville et al., Reference McConville, Saunders, Kloehn and Dagley2015). The glucose-sparing metabolism of the intracellular amastigotes questions the importance of oxidative phosphorylation, and hence ATP production by the ATP synthase, for the parasite. Nevertheless, amastigotes show increased reliance on mitochondrial metabolism, including tricarboxylic acid (TCA) cycle and fatty acid β-oxidation (Saunders et al., Reference Saunders, Ng, Kloehn, Chambers, Ng and McConville2014). These pathways generate reduced electron carriers NADH and FADH2 that are reoxidized by the ETC coupled to ATP synthase (Dey et al., Reference Dey, Meneses, Salotra, Kamhawi, Nakhasi and Duncan2010; Saunders et al., Reference Saunders, Ng, Kloehn, Chambers, Ng and McConville2014). The ablation of aconitase, a TCA cycle enzyme, cytochrome c oxidase, or ATP synthase is predicted to be lethal by an in silico-generated model of the metabolic network (Subramanian et al., Reference Subramanian, Jhawar and Sarkar2015). Indeed, the inhibition of ATP synthase by the cell-permeable antimicrobial peptide histatin 5 arrests of Leishmania amastigotes' growth (Luque-Ortega et al., Reference Luque-Ortega, van't Hof, Veerman, Saugar and Rivas2008). Nevertheless, to prove that oxidative phosphorylation is critical for the Leishmania amastigote forms, further studies are necessary (Fig. 4).
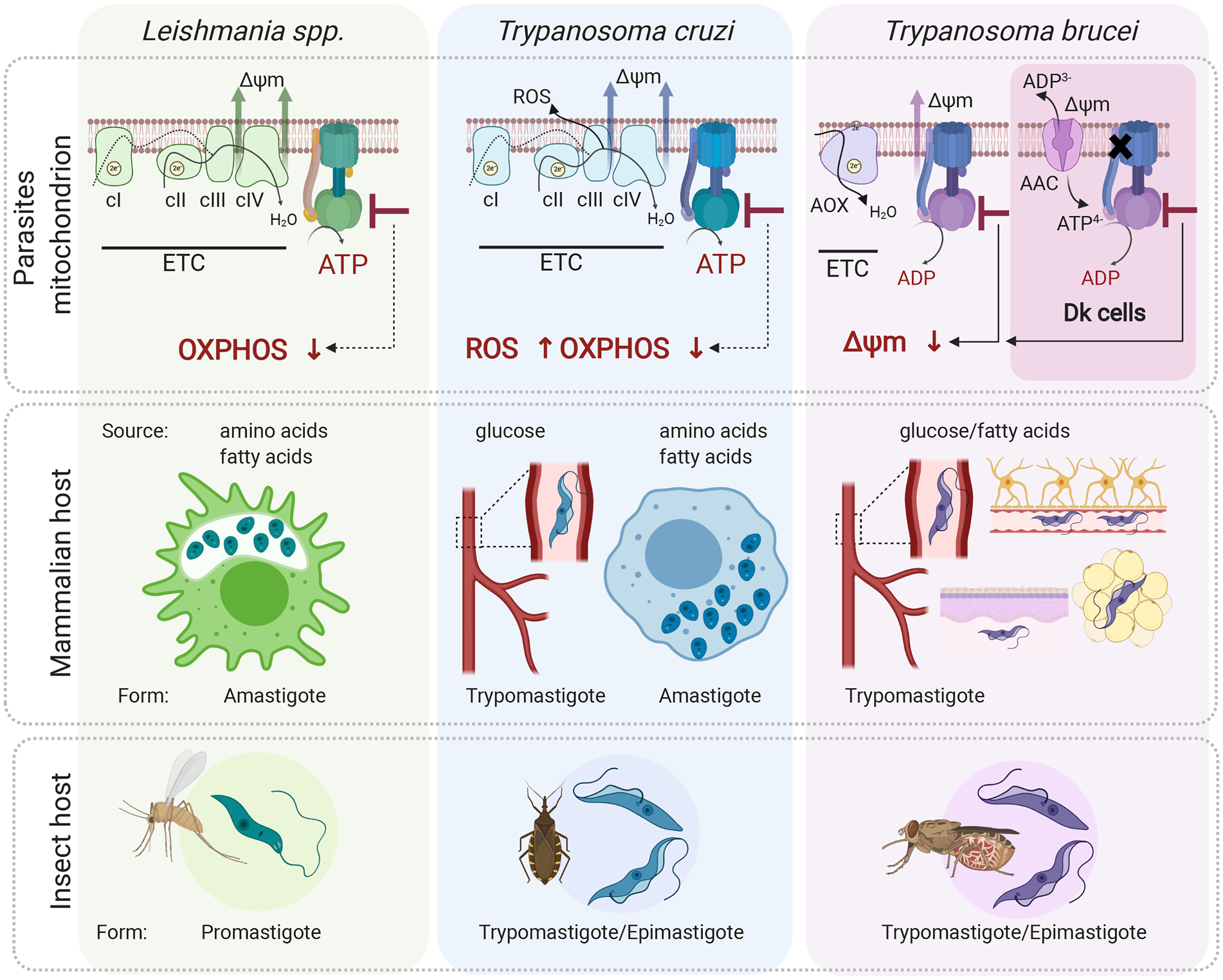
Fig. 4. Role of the mitochondrial ATP synthase in Leishmania, Trypanosoma cruzi and T. brucei disease-causing life cycle stages. In the mammalian host, Leishmania parasites proliferate in macrophages as intracellular amastigotes, while T. cruzi parasites exist in two different forms; as intracellular amastigotes of various mammalian cells and as extracellular trypomastigotes in the host bloodstream. In these life forms, ATP synthase is involved in oxidative phosphorylation and generates ATP. Its inhibition is predicted to cause a depletion of cellular ATP levels. Interestingly, in T. cruzi trypomastigotes, the ATP synthase inhibition would also lead to increased levels of ROS due to differential expression of ETC complexes III and IV. Trypanosoma brucei parasites proliferate in the mammalian bloodstream but also invade interstitial spaces of various tissues such as brain, adipose tissue and skin. Importantly, T. brucei ATP synthase works in reverse and maintains mitochondrial membrane potential (Δψ m) even in trypanosomes lacking mitochondrial DNA. These Dk parasites employ vestigial ATP synthase coupled to ATP/ADP carrier (AAC) to generate Δψ m electrogenically. Inhibition of F-ATPase in both forms leads to dissipation of Δψ m and cell death. All three aforementioned parasites (except for Dk T. brucei) possess a digenetic life cycle involving insect vectors, namely sand fly for Leishmania, triatomine bug for T. cruzi and tsetse fly for T. brucei transmission. Figure created with Biorender.com.
Trypanosoma cruzi parasites are transmitted to humans by a blood-sucking triatomine bug through contact with its infected feces. In the skin wound, metacyclic trypomastigotes invade various types of cells, escape the lysosome-derived parasitophorous vacuole and establish infection in the host cell cytoplasm as intracellular amastigotes (de Souza et al., Reference de Souza, de Carvalho and Barrias2010). During T. cruzi trypomastigotes' transition to amastigotes, there is a dramatic shift from sugar-based metabolism to catabolism of amino and fatty acids manifested by repression of glucose transporters and increased levels of TCA cycle enzymes and proteins involved in fatty acid oxidation and oxidative phosphorylation. The oxidative metabolism of amino and fatty acids suggests a crucial role for oxidative phosphorylation to generate ATP (Atwood et al., Reference Atwood, Weatherly, Minning, Bundy, Cavola, Opperdoes, Orlando and Tarleton2005; Silber et al., Reference Silber, Tonelli, Lopes, Cunha-e-Silva, Torrecilhas, Schumacher, Colli and Alves2009; Li et al., Reference Li, Shah-Simpson, Okrah, Belew, Choi, Caradonna, Padmanabhan, Ndegwa, Temanni, Corrada Bravo, El-Sayed and Burleigh2016; Shah-Simpson et al., Reference Shah-Simpson, Pereira, Dumoulin, Caradonna and Burleigh2016). This is further supported by the identification of highly selective compounds (i.e. GNF7686 and ELQ271) that target complex III and inhibit the growth of intracellular amastigotes in a dose-dependent manner (Khare et al., Reference Khare, Roach, Barnes, Hoepfner, Walker, Chatterjee, Neitz, Arkin, McNamara, Ballard, Lai, Fu, Molteni, Yeh, McKerrow, Glynne and Supek2015).
After several rounds of intracellular replication, amastigotes differentiate to trypomastigotes, which are released from the cell by its rupture. Extracellular trypomastigotes disseminate and infect other cells or they are ingested by the triatomine bug to conclude the life cycle. Interestingly, the extracellular bloodstream trypomastigotes switch back to glycolysis to meet their energy demands but still employ ETC complexes to maintain the mitochondrial membrane potential. Moreover, disproportional changes in expression of ETC complexes II, III and IV create a bottleneck forcing electrons to prematurely reduce molecules of oxygen and generate significant levels of ROS (Goncalves et al., Reference Goncalves, Barreto, Polycarpo, Gadelha, Castro and Oliveira2011). This induced production of ROS probably provides a redox-mediated pre-conditioning to evoke protection against host-induced oxidative challenge once the parasite enters the cell again. In this particular case, the ETC linked to ATP synthase also serves as an adaptive process allowing parasites to survive redox challenges imposed by the host. Presumably, inhibition of the ATP synthase would cause a decrease in ATP levels, which by itself can be lethal, but it would also further increase the already elevated ROS levels. Although there is evidence that the oxidative environment stimulates the parasite's growth and ROS are critical for successful infection of T. cruzi in various cell types (Paiva and Bozza, Reference Paiva and Bozza2014), very high levels of ROS reverse this favourable effect and are harmful for the parasite (Goes et al., Reference Goes, Rocha, Diniz, Aguiar, Machado and Vieira2016). Therefore, an additive effect of endogenous and exogenous ROS bursts may induce deleterious changes resulting in parasites' elimination by oxidative damage (Fig. 4).
Despite some evidence that mitochondrial ETC and ATP synthase might be essential entities for the clinically relevant infectious stages of Leishmania and T. cruzi parasites, the knowledge of the mitochondrial energy metabolism in these parasites remains largely incomplete.
The F-ATPase in the bloodstream form of T. brucei
The disease-causing form of T. brucei is an extracellular parasite inhabiting various niches of its mammalian host, including the bloodstream, adipose tissue, skin or central nervous system (Silva Pereira et al., Reference Silva Pereira, Trindade, De Niz and Figueiredo2019). Since these environments offer different nutrients, the BSF mitochondrion maintains its complexity and plasticity despite its largely reduced size compared to the insect stage organelle (Zikova et al., Reference Zikova, Verner, Nenarokova, Michels and Lukes2017). Uniquely, the parasite's mitochondrion employs ATP synthase as an ATP-consuming proton pump (Fig. 4).
The discovery of the oligomycin-sensitive F-ATP synthase in BSF by Opperdoes et al. (Reference Opperdoes, Borst and Spits1977), elicited the curiosity of scientists, who back then considered the BSF mitochondrion a vestigial organelle with no obvious role in cellular metabolism. Seeking if this ‘promitochondrion’ possesses mitochondrial membrane potential, Nolan and Voorheis reported the existence of electrochemical gradients in BSF trypanosomes, documented by the accumulation of the radiolabelled lipophilic cation methyltriphenylphosphonium. One year after, the same authors reported that oligomycin caused a collapse of the mitochondrial membrane potential identical in magnitude to that achieved by the proton uncoupler FCCP, concluding that in BSF cells, the nature of the mitochondrial electrical gradient was exclusively attributable to the proton-pumping activity, or reverse mode, of the ATP synthase (Nolan and Voorheis, Reference Nolan and Voorheis1992). In more than 10 years, RNA interference silencing of two F1-ATPase subunits further corroborated the previous findings and confirmed the unusual and essential function of this enzyme for the survival of BSF trypanosomes (Schnaufer et al., Reference Schnaufer, Clark-Walker, Steinberg and Stuart2005; Brown et al., Reference Brown, Hosking, Li and Williams2006).
The role of the F-ATPase in BSF T. brucei strikingly contrasts with that of other eukaryotes, where the reverse operation of the ATP synthase is a rare and short-term phenomenon associated with hypoxia or anoxia. For example, under ischaemic conditions in mammalian tissues, respiration is halted and the ATP synthase reverses in response to decreased mitochondrial membrane potential, partially compensating for its loss by utilizing ATP produced by glycolysis. To limit the detrimental depletion of cellular ATP caused by an ATP-consuming F-ATP synthase (Rouslin et al., Reference Rouslin, Erickson and Solaro1986; Jennings et al., Reference Jennings, Reimer and Steenbergen1991; St-Pierre et al., Reference St-Pierre, Brand and Boutilier2000), the activity of this enzyme is regulated by a short naturally occurring protein, the inhibitory factor 1 (IF1) (Pullman and Monroy, Reference Pullman and Monroy1963; Walker, Reference Walker1994). IF1 specifically inhibits the ATPase activity with no effect on ATP synthesis (Pullman and Monroy, Reference Pullman and Monroy1963; Asami et al., Reference Asami, Juniti and Ernster1970), although some authors propose an inhibitory effect of IF1 on both ATP synthase modes (Harris et al., Reference Harris, von Tscharner and Radda1979; Schwerzmann and Pedersen, Reference Schwerzmann and Pedersen1981; Formentini et al., Reference Formentini, Sanchez-Arago, Sanchez-Cenizo and Cuezva2012; Garcia-Bermudez and Cuezva, Reference Garcia-Bermudez and Cuezva2016). IF1 has also been implicated in cristae biogenesis, dimerization of the ATP synthase and metabolic reprogramming of cancer cells (Campanella et al., Reference Campanella, Parker, Tan, Hall and Duchen2009). This regulatory protein has been identified in T. brucei (TbIF1) and related trypanosomatid parasites. In T. brucei the expression of TbIF1 is tightly regulated throughout the life cycle as it is expressed only in the insect stages, but not in BSF (Panicucci et al., Reference Panicucci, Gahura and Zikova2017). In T. cruzi and Leishmania parasites, regulation of IF1 expression is reflected in its slight upregulation in the mammalian intracellular stages compared to the respective insect stages in agreement with increased mitochondrial activity of T. cruzi and Leishmania amastigotes (Li et al., Reference Li, Shah-Simpson, Okrah, Belew, Choi, Caradonna, Padmanabhan, Ndegwa, Temanni, Corrada Bravo, El-Sayed and Burleigh2016; Inbar et al., Reference Inbar, Hughitt, Dillon, Ghosh, El-Sayed and Sacks2017). As in other organisms, TbIF1 inhibits the hydrolytic but not the synthetic activity of the mitochondrial ATP synthase in vitro (Panicucci et al., Reference Panicucci, Gahura and Zikova2017; Gahura et al., Reference Gahura, Panicucci, Vachova, Walker and Zikova2018a). The artificial expression of TbIF1 is lethal for BSF trypanosomes, due to the abolishment of the mitochondrial membrane potential (Panicucci et al., Reference Panicucci, Gahura and Zikova2017).
In addition to its role in the maintenance of mitochondrial membrane potential, a functional F-ATPase is also required by BSF parasites to prevent the intramitochondrial accumulation of ATP. Elevated ATP levels lead to inhibition of the alternative oxidase, the only terminal oxidase in BSF parasites, and consequently to a reduced respiration rate (Luevano-Martinez et al., Reference Luevano-Martinez, Girard, Alencar and Silber2020). This represents a novel and unique role of the F-ATPase connecting this enzyme to the regulation of the glycolytic pathway, which is intimately linked to respiration via the alternative oxidase (Bakker et al., Reference Bakker, Michels, Opperdoes and Westerhoff1999). Despite these fundamental roles, BSF trypanosomes can withstand a loss of approximately 90% of the membrane-bound F-ATPase complexes with no obvious deleterious effect on their viability in culture nor on the mitochondrial membrane potential in live cells (Hierro-Yap et al., Reference Hierro-Yap, Subrtová, Gahura, Panicucci, Dewar, Chinopoulos, Schnaufer and Zikova2021). We speculate that the long-term tolerance of reduced levels of F-ATPase complexes might be favourable for the emergence of trypanosomes lacking mitochondrial genome, as it might provide the time frame for the parasites to gain key nuclear mutation(s) in the F-ATPase that enable(s) generation of mitochondrial membrane potential in the absence of mitochondrial-encoded a-subunit.
The vestigial F-ATPase in trypanosomes without mitochondrial genome
The mitochondrial DNA of trypanosomatids, which is arranged in a distinct submitochondrial structure termed kinetoplast (hence kinetoplast DNA, kDNA), encodes mostly for the components of the oxidative phosphorylation pathway, key for the survival of T. brucei's insect stages (Stuart, Reference Stuart1983; Schnaufer et al., Reference Schnaufer, Domingo and Stuart2002). In BSF trypanosomes, which do not carry out oxidative phosphorylation, kDNA is indispensable due to the requirement of the F-ATP synthase a-subunit involved in proton translocation across the inner mitochondrial membrane. To synthesize the a-subunit, BSF mitochondria express additional two kDNA-encoded proteins, uS3m and uS12m, core components of mitochondrial ribosomes (Ramrath et al., Reference Ramrath, Niemann, Leibundgut, Bieri, Prange, Horn, Leitner, Boehringer, Schneider and Ban2018). However, there are BSF trypanosomes that partially or completely lack their kDNA and they are referred to as dyskinetoplastic (Dk) subspecies (Agbe and Yielding, Reference Agbe and Yielding1995). The Dk trypanosomes thriving in nature, T. brucei evansi (Hoare, Reference Hoare1937) and T. brucei equiperdum (Tobie, Reference Tobie1951), cause surra and dourine, respectively, devastating diseases that affect a broad range of domestic and wild animals in Africa, Asia and South America (Brun et al., Reference Brun, Hecker and Lun1998). Stable kDNA-deficient strains can also be generated artificially using compounds targeting the kDNA network, such as ethidium bromide or acriflavine (Stuart, Reference Stuart1971; Riou et al., Reference Riou, Belnat and Benard1980; Riou and Benard, Reference Riou and Benard1980). Although Dk trypanosomes were thought to be locked in the long slender BSF stage, the absence of kDNA does not impair their differentiation into the stumpy form, a transmission pre-adapted stage with partially activated mitochondrial functions. However, the absence of kDNA does reduce the lifespan of the stumpy form (Dewar et al., Reference Dewar, MacGregor, Cooper, Gould, Matthews, Savill and Schnaufer2018), hampering their differentiation into the procyclic form (Timms et al., Reference Timms, van Deursen, Hendriks and Matthews2002). Therefore, Dk trypanosomes are transmitted between hosts during sexual intercourse or mechanically via blood-sucking insects or vampire bats (Brun et al., Reference Brun, Hecker and Lun1998), which facilitated their spread outside the area of the tsetse fly belt (Lun and Desser, Reference Lun and Desser1995).
Because the ATP synthase a-subunit is absent in Dk trypanosomes, the molecular mechanism for the generation of mitochondrial membrane potential is analogous to that of mammalian ρ 0 cells and yeast petite mutants, both of which lack mitochondrial genome. The mechanism involves ATP hydrolysis by the vestigial ATP synthase, which provides the substrate for the electrogenic exchange of mitochondrial ADP3− for cytosolic ATP4− by the ATP/ADP carrier (AAC). Accordingly, oligomycin, an inhibitor that blocks the c-ring rotation, has virtually no effect on the growth of T. b. evansi (Schnaufer et al., Reference Schnaufer, Clark-Walker, Steinberg and Stuart2005). Moreover, Dk strains contain one of several point mutations in the nuclear-encoded subunits of the F1-ATPase, most often the L262P or A273P substitutions in the γ-subunit (Lai et al., Reference Lai, Hashimi, Lun, Ayala and Lukes2008; Dean et al., Reference Dean, Gould, Dewar and Schnaufer2013). These substitutions are sufficient to allow the loss of kDNA and enable the generation of mitochondrial membrane potential in an F0-independent manner, perhaps by enhancing the hydrolytic activity of the F1-ATPase (Dean et al., Reference Dean, Gould, Dewar and Schnaufer2013).
The functional independence from the F0 section led to the long-standing belief that the F1 moiety exists as a soluble entity detached from the inner mitochondrial membrane in Dk trypanosomes (Schnaufer et al., Reference Schnaufer, Clark-Walker, Steinberg and Stuart2005; Jensen et al., Reference Jensen, Simpson and Englund2008). However, the finding that the silenced expression of the peripheral stalk subunit ATPTB2/d-subunit slowed the growth of Dk trypanosomes in vitro (Subrtova et al., Reference Subrtova, Panicucci and Zikova2015) indicates that the complex might be attached to the membrane. Considering the presence of a membrane-bound F-ATP synthase in cells devoid of mitochondrial genome in other organisms (Wittig et al., Reference Wittig, Meyer, Heide, Steger, Bleier, Wumaier, Karas and Schagger2010; He et al., Reference He, Carroll, Ding, Fearnley and Walker2017), it is likely that the vestigial F-ATPase in Dk trypanosomes also remains associated with the membrane to increase the spatial proximity between the AAC and the F1-ATPase, and ensure an efficient exchange of substrates between the two entities.
Perspectives
Although many architectural features of ATP synthase from Euglena can be extrapolated to Trypanosomatida, a high-resolution cryo-EM structure of a trypanosomatid ATP synthase will be necessary for an unbiased and complete description of the enzyme in this parasitic lineage. Such research will reveal structural divergency, predominantly in the membrane-embedded part of the enzyme, as suggested by the absence of apparent homologs of some conserved ATP synthase subunits in T. brucei (Fig. 1). If several rotational states of the enzyme are characterized, the study would explain how the apparently flexible peripheral stalk immobilizes the catalytic subcomplex and what is the contribution of its unprecedented contacts with the F1-ATPase (Fig. 3D). An atomic model of trypanosomal ATP synthase could also reveal details with potential relevance to structure-based drug development. For example, the IF1-F1-ATPase interaction interface can be exploited by peptidomimetics to develop inhibitors selective for ATP hydrolytic activity of the enzyme. In the insect stage of T. brucei, the dimers of ATP synthase contribute to the shaping of the inner mitochondrial membrane by assembling into short helices on the rims of discoidal cristae (Muhleip et al., Reference Muhleip, Dewar, Schnaufer, Kuhlbrandt and Davies2017). However, it is not clear how the dimers arrange in the BSF cells, which exhibit reduced complexity of mitochondrial ultrastructure. The understanding of the molecular mechanisms of membrane reshaping during the T. brucei life cycle, which can now be mimicked in vitro (Kolev et al., Reference Kolev, Ramey-Butler, Cross, Ullu and Tschudi2012; Dolezelova et al., Reference Dolezelova, Kunzova, Dejung, Levin, Panicucci, Regnault, Janzen, Barrett, Butter and Zikova2020), will further illuminate the principles underlying mitochondrial dynamics in eukaryotes in general. Furthermore, recent data suggested interaction of the ATP synthase complex with mitochondrial calcium uniporter complex, AAC and phosphate carrier (Huang and Docampo, Reference Huang and Docampo2020). Further studies scrutinizing this putative megacomplex may reveal ATP synthase regulation by Ca2+ and strengthen the importance of the functional interplay between ATP synthase and both carriers in BSF and Dk cells. Considering our detailed knowledge of ATP synthase's role in T. brucei parasites, it is surprising that very little is known about its function in medically important parasites, Leishmania and T. cruzi. Although this gap is caused most likely by the fact that these parasites are less amenable to genetic manipulation, this can now be overcome by using subgenomic CRISPR/Cas9 libraries to dissect the role of oxidative phosphorylation complexes in different life cycle forms of these parasites. This will lead to a better understanding of the parasites' metabolic flexibility and capacity, ultimately advancing our efforts to target mitochondrial essential metabolic pathways for drug development.
Financial support
This study was supported by the Czech Science Foundation (18-17529S) and ERD fund and Ministry of Education, Youth and Sports (CZ.02.1.01/0.0/0.0/16_019/0000759).
Conflict of interest
The authors declare no competing interests.