INTRODUCTION
Organic carbon (C) is reduced C whose molecular structure is formed on skeletons of C atoms. There are two pools of organic C in seawater, dissolved organic C (DOC) that passes a 0.2 to 1.0 micron filter, and particulate organic C (POC) that is retained on the filter (Figure 1). The pool size of DOC is 682 GtC (Hansell Reference Hansell2013), similar in size to the organic C present in the upper one meter of oceanic sediments (1000 GtC, Hedges et al. Reference Hedges2000); the POC pool is much smaller (30–40 GtC). Early studies demonstrated that bomb 14C was incorporated into subsurface and deep organisms (technically a portion of the POC pool), such as decapods and fish, along with significant amounts of pre-bomb C, on decadal timescales (Williams et al. Reference Williams, McGowan and Stuiver1970; Williams and Linick Reference Williams and Linick1975).

Figure 1 Size ranges of organic matter constituents in seawater and their relationships to the operationally defined pools of DOC (gray background) and POC (white background). (After Beaupré Reference Ausín, Magill, Haghipour, Fernández, Wacker, Hodell, Baumann and Eglinton2019.)
Organic C is primarily produced during photosynthetic reduction of dissolved inorganic C (DIC) by phototrophs in the surface ocean. The concentration of DOC in seawater is 2–4% that of DIC, which makes DOC more sensitive to changes in its sources and sinks than those for DIC. The processes controlling DIC in the ocean are CO2 exchange with the atmosphere, photosynthesis, respiration and production and dissolution of carbonate. The processes controlling DOC in seawater are more complex, including river input, microbial production of recalcitrant DOC (Jiao et al. Reference Jiao, Herndl, Hansell, Benner, Kattner, Wilhelm, Kirchman, Weinbauer, Chen and Azam2010), chemoautotrophy (Ingalls et al. Reference Ingalls, Shah, Hansman, Aluwihare, Santos, Druffel and Pearson2006; Hansman et al. Reference Hansman, Griffin, Watson, Druffel, Ingalls, Pearson and Aluwihare2009), hydrothermal processes (McCarthy et al. Reference McCarthy, Beaupré, Walker, Voparil, Guilderson and Druffel2011; Lang et al. Reference Lang, Butterfield, Lilley, Paul Johnson and Hedges2006; Shah Walter et al. Reference Shaw Walter, Jaekel, Osterholz, Fisher, Huber and Pearson2018; Estes et al. Reference Estes, Berti, Coffey, Hochella, Wozniak and Luther2019), enhanced refractory DOC utilization by priming (Bianchi et al. Reference Bianchi2011; Fang et al. Reference Fang, Lee, Lee, Hahm, Kim, Druffel and Hwang2020; Shen and Benner Reference Shen and Benner2018), gel formation (Verdugo et al. Reference Verdugo, Alldredge, Azam, Kirchmand, Passowe and Santschif2004) and dissolution/aggregation of POC (Smith et al. Reference Smith, Baldwin and Williams1992; Druffel et al. Reference Druffel, Griffin, Coppola and Walker2016).
The introduction of 14C analyses using AMS in the 1980s made it possible to study a broad range of organic C pools in the sea that were not possible with gas counting or liquid scintillation methods due to sample size restrictions. We discuss the present and developing advances for studying marine organic C using the mother of all isotopes, 14C.
Bulk DOC 14C
Methods for measuring bulk DOC Δ14C in seawater were developed in the 1960s that required collection of 400–500 L of water in Gerard barrels (Figure 2), UV oxidation in 60 L batches, and gas counting of 14C in the CO2 (Williams et al. Reference Williams, Oeschger and Kinney1969). Improvements to the method required UV oxidation of 5 L of seawater using AMS measurements of 14C (Williams and Druffel Reference Williams and Druffel1987). Beaupré et al. (Reference Beaupré, Druffel and Griffin2007) improved the method further by using UV oxidation of samples as small as 30 mL of seawater. Quantification of CO2 lost during breakthrough of CO2 from liquid nitrogen cooled traps during collection improved DOC concentrations reported with isotopic values (Walker et al. Reference Walker, Beaupré, Griffin and Druffel2019). However, one day is required to process one sample, standard, or blank. New methods are critically needed to increase processing of DOC samples for 14C measurement. Batch processing of bulk DOC in 100 mL reactors improves throughput of high [DOC] samples, such as freshwater and sediment pore water, to several per day (Xue et al. Reference Xue, Tiantian and Xuchen2015).

Figure 2 Peter M. Williams on the R/V Melville with a Gerard barrel, a 270 L steel vessel used to collect large volume seawater samples. The barrel is covered to protect it from particles coming mostly from the ship’s stack. Circa 1989. (Photo by Ellen Druffel.)
Radiocarbon in DIC has been used to measure the timescale of global deep ocean circulation. Deep water is produced in the northern North Atlantic, flows south to the Southern Ocean where it flows eastward, and then northward into the Indian and Pacific oceans, taking ∼1500 14C years to complete its journey (Broecker et al. Reference Broecker, Gerard, Ewing and Heezen1960; Bien et al. Reference Bien, Rakestraw and Suess1965; Stuiver et al. Reference Stuiver, Quay and Ostlund1983). This result agrees with pre-bomb bulk DOC Δ14C values that decrease from the deep North Atlantic (–456‰, 4900 14C years), to the South Atlantic (–475‰, 5200 14C years), the Southern Ocean (–500‰, 5600 14C years), and are lowest in the North Pacific (–560‰, 6600 14C years) (Druffel et al. Reference Druffel, Griffin, Coppola and Walker2016, Reference Druffel, Griffin, Garcia, Wang, McNichol, Key and Walker2019; Bercovici et al. Reference Bercovici, McNichol, Xu and Hansell2018; Druffel and Bauer Reference Druffel and Bauer2000), increasing in 14C age by 1700 years. This remarkable similarity between the deep cycling time of DOC and DIC suggests that DOC Δ14C is controlled primarily by circulation, indicating that deep DOC is refractory on millennial timescales (Hansell Reference Hansell2013). Yet the DOC 14C ages are much lower (∼3000 14C years) than those of DIC.
There are significant correlations between DOC and DIC Δ14C values for seawater taken from the same niskin bottle at numerous locations in the world oceans (Beaupré and Aluwihare Reference Beaupré and Aluwihare2010; Beaupré et al. Reference Beaupré, Walker and Druffel2020). Similar to DIC Δ14C results in the central Pacific (<1,000 m) that contained bomb 14C in the 1980s (Ostlund and Stuiver Reference Ostlund and Stuiver1980) and >1990s (Key et al. Reference Key, Kozyr, Sabine, Lee, Wanninkhof and Bullister2004; McNichol et al. Reference McNichol, Key and Guilderson2022), bomb 14C is seen in the DOC Δ14C values in the upper 1000 m in the Pacific Ocean (Figure 3). The highest surface values are found in the mid-ocean gyres around 20°N and 20°S (>–250‰), and lower values are near the equator and polar regions (–260‰ to –400‰) where deeper waters upwell to the surface (Bercovici et al. Reference Bercovici, McNichol, Xu and Hansell2018; Druffel and Bauer Reference Druffel and Bauer2000). Subantarctic Mode Water, produced in the southwest Pacific, flows between 200 and 400 m to the Equatorial Undercurrent in the equatorial Pacific, and has Δ14C values that range from –460 to –350‰ (Figure 3). Formed between 60° and 50°S, Antarctic Intermediate Water flows north at depths of ∼700–1200 m and has Δ14C values that range from –530 to –410‰ (Figure 3).

Figure 3 DOC Δ14C values (‰) of water samples (indicated by black dots) collected from seven stations along 150°W on the P16N cruise in 2015 (Druffel et al. Reference Druffel, Griffin, Garcia, Wang, McNichol, Key and Walker2019), one station on the P06 cruise (stn 130, 32.5°S 144.7°W) in 2010 (Druffel and Griffin Reference Druffel and Griffin2015) and one station from the Southern Ocean (SOce; 54.0°S 176.0°W) in 1995 (Druffel and Bauer Reference Druffel and Bauer2000) using Ocean Data View (Schlitzer Reference Schlitzer2015). (After Druffel et al. Reference Druffel, Griffin, Garcia, Wang, McNichol, Key and Walker2019.)
Questions that remain unanswered about marine DOC include the sources and sinks of DOC from hydrothermal systems, chemoautotrophy, sediment porewaters, interaction with POC, and photooxidation of DOC in the surface ocean and aerosols (Beaupré et al. Reference Beaupré, Walker and Druffel2020). 14C and molecular identification methods (e.g., NMR and FT-ICR-MS) can help to determine the importance of these mechanisms.
SPE-DOC, and Size-Fractionated DOC
Solid-phase extraction (SPE) is a method of separating DOC (SPE-DOC) from bulk DOC using hydrophobic, silica or polystyrene resins. Seawater is acidified to enhance the relative abundance of protonated carboxyl groups, decreasing solubility and increasing sorption onto the resin (Mopper et al. Reference Mopper, Stubbins, Ritchie, Bialk and Hatcher2007). XAD and C18 resins have been used to collect SPE-DOC and generally isolate humic substances from total DOC (Druffel et al. Reference Druffel, Williams, Bauer and Ertel1992; Green and Blough Reference Green and Blough1994; Hedges Reference Hedges1992; Kieber et al. Reference Kieber, Hydro and Seaton1997; Peuravuori and Pihlaja Reference Peuravuori and Pihlaja1997). C18 resins were especially effective in isolating chromophoric humic substances (Amador et al. Reference Amador, Milne, Moore and Zika1990). More recently, styrene divinylbenzene polymer sorbents, such as PPL are more efficient at extracting DOC and leach less C than C18 resins. NMR analysis shows that SPE-DOC isolated using PPL is more representative of bulk DOC than that isolated using C18 resins (Dittmar et al. Reference Dittmar, Koch, Hertkorn and Kattner2008). The ease of use, high recoveries, and ability to desalinate SPE-DOC samples have led to wide use of PPL resins in investigations of marine DOC. Subsequent methodological studies have compared PPL, C18, and other SPE resins in more detail, and show that DOC extraction can be increased up to 89% with the correct ratio of DOC/PPL (Li et al. Reference Li, Harir, Lucio, Kanawati, Smirnov, Flerus, Koch, Schmitt-Kopplin and Hertkorn2016). Lewis et al. (Reference Lewis, Walker and Druffel2020) demonstrated that the optical and isotopic characteristics of SPE-DOC using PPL cartridges are heterogeneous during elution with methanol, which may be useful in further separation of compounds based on their relative polarities.
PPL has been used to isotopically and chemically characterize SPE-DOC in the east South Atlantic Ocean, the Bermuda Atlantic Time Series, Station ALOHA in the North Pacific, the Weddell Sea, and the deep North Pacific Ocean (Broek et al. Reference Broek, Walker, Guilderson, Vaughn, Mason and McCarthy2020; Flerus et al. Reference Flerus, Lechtenfeld, Koch, McCallister, Schmitt-Kopplin, Benner, Kaiser and Kattner2012; Hertkorn et al. Reference Hertkorn, Harir, Koch, Michalke and Schmitt-Kopplin2013; Lechtenfeld et al. Reference Lechtenfeld, Kattner, Flerus, McCallister, Schmitt-Kopplin and Koch2014; Zigah et al. Reference Zigah, McNichol, Xu, Johnson, Santinelli, Karl and Repeta2017). SPE-DOC typically has lower Δ14C and δ13C values than those of total DOC (Broek et al. Reference Broek, Walker, Guilderson, Vaughn, Mason and McCarthy2020; Coppola and Druffel Reference Coppola and Druffel2016; Zigah et al. Reference Zigah, McNichol, Xu, Johnson, Santinelli, Karl and Repeta2017; Lewis et al. Reference Lewis, Walker and Druffel2021), is rich in aromatic C (Simjouw et al. Reference Simjouw, Minor and Mopper2005), and has elemental compositions indicative of thermogenic processes found throughout the water column (Dittmar and Koch Reference Dittmar and Koch2006; Hertkorn et al. Reference Hertkorn, Harir, Koch, Michalke and Schmitt-Kopplin2013). The presence of carboxyl-rich alicyclic molecules (CRAM), with low H/C and O/C ratios indicative of recalcitrance, is often found in SPE-DOC and tends to increase with depth in the water column (Broek et al. Reference Broek, Walker, Guilderson, Vaughn, Mason and McCarthy2020; Hertkorn et al. Reference Hertkorn, Harir, Koch, Michalke and Schmitt-Kopplin2013; Lechtenfeld et al. Reference Lechtenfeld, Hertkorn, Shen, Witt and Benner2015). Lechtenfeld (Reference Lechtenfeld, Hertkorn, Shen, Witt and Benner2015) found that bacterial SPE-DOC isolated in laboratory experiments is similar in composition with natural marine DOC and the composition of recalcitrant molecules in the ocean. The link between SPE-DOC recalcitrance, low Δ14C, and low δ13C may all be indicative of the influence of marine heterotrophic bacteria on DOC degradation, transformation, cycling, and the production of refractory DOC (Flerus et al. Reference Flerus, Lechtenfeld, Koch, McCallister, Schmitt-Kopplin, Benner, Kaiser and Kattner2012; Lechtenfeld et al. Reference Lechtenfeld, Hertkorn, Shen, Witt and Benner2015; Osterholz et al. Reference Osterholz, Niggemann, Giebel, Simon and Dittmar2015). Solid-phase extraction will likely continue to advance our knowledge of DOC molecular composition as techniques to isolate and further characterize DOC develop further.
For several decades, ultrafiltration (UF) has been used as a method to isolate DOC from abundant salts in seawater. The technique has shaped much of our understanding of DOC cycling and molecular composition, and samples material that can be isolated without the chemical bias inherent to all resin chromatography methods. Early UF studies have shown that, despite low DOC recovery (10–30%), ultrafiltered DOC (UDOC), also referred to as high molecular weight (HMW DOC), was generally representative of total DOC in terms of its bulk elemental (C:N) and isotopic (δ13C, δ15N) values (Benner et al. Reference Benner, Paculksy, McCarthy and Hedges1992, Reference Benner, Biddanda, Black and McCarthy1997; McCarthy et al. Reference McCarthy, Hedges and Benner1993). However, several studies have shown that UDOC differs from bulk DOC in molecular composition (Skoog and Benner Reference Skoog and Benner1997; Dittmar et al. Reference Dittmar, Fitznar and Kattner2001) and Δ14C values (Santschi et al. Reference Santschi, Guo, Baskaran, Trumbore, Southon, Bianchi, Honeyman and Cifuentes1995; Loh et al. Reference Loh, Bauer and Druffel2004; McNichol and Aluwihare Reference McNichol and Aluwihare2007). Further parameterization of UDOC Δ14C values as a function of UF experiment concentration factor (CF) reconciled >250‰ variability in reported surface and deep Pacific UDOC Δ14C values (Figure 4a; Walker et al. Reference Walker, Beaupre, Guilderson, Druffel and McCarthy2011). Further work suggested that changes in CF could be used for the targeted isolation of semi-labile or even bioavailable UDOC (Walker et al. Reference Walker2016a). Robust, size-age-composition relationships within both the DOC pool and total marine organic matter size continuum (Figure 4b,c,d) suggest a “precursor-product” relationship controlling the cycling (14C age) and chemical composition (N-content) of marine organic matter. As a result, DOC C:N content and 14C age can be generally predicted from molecular size (Walker et al. Reference Walker, Beaupre, Guilderson, McCarthy and Druffel2016b).

Figure 4 Size-Δ14C and elemental composition (C:N) relationships of DOC in the Pacific Ocean. (A) DOC Δ14C as a function of ultrafiltration concentration factor (CF) for the surface and deep Pacific ocean. Relationships between the C:N content of size-fractionated marine organic matter (B,D) and Δ14C (C,E) in the surface and deep Pacific. (Figures adapted from Walker et al. Reference Walker, Beaupre, Guilderson, Druffel and McCarthy2011 and Walker et al. Reference Walker, Beaupre, Guilderson, McCarthy and Druffel2016b.)
A recent advancement is the combination of UF and SPE techniques to view organic matter through various analytical windows simultaneously. By filtering out POC, then performing solid-phase extraction on UDOC permeate fractions, the comparison of up to three different sub-pools of DOC is possible (HMW-DOC, hydrophobic LMW-DOC, hydrophilic LMW DOC) (Broek et al. Reference Broek, Walker, Guilderson and McCarthy2017; Zigah et al. Reference Zigah, McNichol, Xu, Johnson, Santinelli, Karl and Repeta2017; Broek et al. Reference Broek, Walker, Guilderson, Vaughn, Mason and McCarthy2020). This method has provided new insights regarding the cycling of hydrothermal vent DOC in the open ocean, the possible addition of high molecular weight DOC to the deep ocean, and the selective utilization of some LMW-DOC during meridional overturning circulation (Broek et al. Reference Broek, Walker, Guilderson and McCarthy2017; Zigah et al. Reference Zigah, McNichol, Xu, Johnson, Santinelli, Karl and Repeta2017; Broek et al. Reference Broek, Walker, Guilderson, Vaughn, Mason and McCarthy2020).
Compound Classes and 14C
Marine organic matter exists as molecules, some of which are characterizable. The characterizable organic C is made up mostly of amino acids/proteins, sugars/carbohydrates, lipids, and nucleic acids. However, more than half of the organic matter in seawater and sediments is not characterizable at the molecular level (Hedges et al. Reference Hedges2000). Whereas 85% of surface plankton and POC in the central Pacific is identified molecularly (Wakeham et al. Reference Wakeham, Lee, Hedges, Hermes and Peterson1997), less than 25% of deep POC is so identified, due to extensive remineralization as POC falls through the water column. The Δ14C of sinking POC in the deep east North Pacific is lower than that of surface DIC that was photosynthesized to produce the organic C (Druffel et al. Reference Druffel, Bauer, Williams, Griffin and Wolgast1996). The surface sediment organic C has even lower Δ14C values (Wang et al. Reference Wang, Druffel and Lee1998). A correlation was found between Δ14C and aluminum content of sinking POC, demonstrating that organic C associated with lithogenic material from sediment resuspension causes the observed low Δ14C values (Hwang et al. Reference Hwang, Druffel and Eglinton2010).
To understand these trends, separation of POC into four compound classes (or organic fractions), total hydrolyzable amino acids (THAA), total hydrolyzable carbohydrates (THCO), lipids and acid insoluble fraction were used to study 14C (Wang et al. Reference Wang, Druffel and Lee1996, Reference Wang, Druffel and Lee1998; Hwang and Druffel Reference Hwang and Druffel2003). Dried POC, zooplankton, phytoplankton, detrital aggregates on surface sediment and sediment from Stn M (34°50ʼN, 123°0ʼW, 4100 m depth) were extracted with methylene chloride:methanol to extract lipids. Half of the residue was hydrolyzed with 6M HCl under N2 for THAA and the other half with 72% and 0.6M H2SO4. The hydrolyzates were eluted through ion exchange columns to separate the two fractions, and the organic C leftover from the THAA extraction was used for the acid insoluble fraction. All THAA and THCO fractions had higher Δ14C values than those for the lipid and acid insoluble fractions (Wang et al. Reference Wang, Druffel and Lee1996, Reference Wang, Druffel and Lee1998; Hwang and Druffel Reference Hwang and Druffel2003) (Figure 5). The proportion of acid insoluble fractions increased with depth in the water column, and was highest in sediment samples (49–69%) and lowest in zooplankton and phytoplankton (<10%) from the upper 100 m. These results implied that the acid insoluble fraction was composed of lipid-like macromolecules.

Figure 5 Δ14C of organic compound classes (THAA, open triangles; TCHO, open circles; lipids, solid triangles; acid insoluble fraction, solid circles). The range of DIC 14C values in surface waters at Stn M ranged from about 40 to 70‰. (After Hwang and Druffel Reference Hwang and Druffel2003.)
Compound classes have also been measured in HMW-DOC. Results similar to those obtained for POC and sedimentary organic C were obtained, where THAA and TCHO had higher Δ14C values than those for lipid and acid insoluble fractions (Loh et al. Reference Loh, Bauer and Druffel2004).
Dissolved black C (DBC) is incompletely combusted organic matter produced from fossil fuel and biomass burning. DBC was measured in HMW-DOC from 4 samples from the Pacific and Atlantic Oceans and found to have Δ14C values that were 470–860‰ lower than those for bulk DOC from the same water (Ziolkowski and Druffel Reference Ziolkowski and Druffel2010). DBC was also measured in SPE-DOC from 6 samples in the Atlantic, Pacific and Arctic Oceans and DBC Δ14C values were 0–560‰ lower than those of SPE-DOC (Coppola and Druffel Reference Coppola and Druffel2016). They concluded that there are two distinct pools of DBC, that in the surface ocean and that in the deep ocean. Coppola et al. (Reference Coppola, Ziolkowski, Masiello and Druffel2014) measured black C in sinking POC and sediments and found that the main transport mechanism of black C to sediments may be sorption of ancient DBC onto POC. Further studies of DBC in marine systems are needed to understand more fully the sources and sinks of DBC and its residence time in the ocean.
Compound Specific Radiocarbon Analyses (CSRA)
Organic matter in the ocean consists of a complex mixture of different compounds, both of terrigenous and marine origin. Greater understanding of C cycling processes in the marine realm can be obtained by isolating specific fractions of marine organic matter that can be ascribed to a known source (biomarkers), by investigating the 14C signature of individual organic compounds. Compound-specific 14C analyses (CSRA) of marine biomarkers have been used to elucidate processes affecting the fate of marine organic matter during transport (e.g., Ohkouchi et al. Reference Ohkouchi, Eglinton, Keigwin and Hayes2002; Mollenhauer et al. Reference Mollenhauer, Eglinton, Ohkouchi, Schneider, Müller, Grootes and Rullkötter2003, Reference Mollenhauer, Inthorn, Vogt, Zabel, Sinninghe Damsté and Eglinton2007; Ausín et al. Reference Ausín, Magill, Haghipour, Fernández, Wacker, Hodell, Baumann and Eglinton2019), the reactivity of different types of marine organic compounds (e.g., Shah et al. Reference Shah, Mollenhauer, Ohkouchi, Eglinton and Pearson2008; Kusch et al. Reference Kusch, Ogawa, Altabet, Butzin, Friedrich, Ohkouchi and Mollenhauer2010, Reference Kusch, Rethemeyer, Hopmans, Wacker and Mollenhauer2016), and C sources utilized for biosynthesis (Ingalls et al. Reference Ingalls, Shah, Hansman, Aluwihare, Santos, Druffel and Pearson2006; Hansmann et al. Reference Hansman, Griffin, Watson, Druffel, Ingalls, Pearson and Aluwihare2009). Improvement of stratigraphies for sediments that are otherwise difficult to date have also relied on CSRA of marine organic compounds (Ingalls et al. Reference Ingalls, Anderson and Pearson2004; Ohkouchi and Eglinton Reference Ohkouchi and Eglington2008). These studies have revealed the long-term residence of marine organic matter in continuous re-suspension loops (e.g., Mollenhauer et al. Reference Mollenhauer, Inthorn, Vogt, Zabel, Sinninghe Damsté and Eglinton2007, Reference Mollenhauer, McManus, Wagner, McCave and Eglinton2011), which has also been shown for terrigenous biomarkers (e.g., Bröder et al. Reference Bröder, Tesi, Andersson, Semiletov and Gustafsson2018; Wei et al. Reference Wei, Mollenhauer, Hefter, Kusch, Grotheer, Schefuß and Jia2021), its preservation in association with fine-grained lithogenic material resulting in long-distance lateral transport and the millennial scale duration of these transport processes (Ohkouchi et al. Reference Ohkouchi, Eglinton, Keigwin and Hayes2002; Mollenhauer et al. Reference Mollenhauer, Kienast, Lamy, Meggers, Schenider, Hayes and Eglinton2005, Reference Mollenhauer, Inthorn, Vogt, Zabel, Sinninghe Damsté and Eglinton2007). Chemoautotrophic metabolism has been shown to prevail among mesopelagic microbial communities (Ingalls et al. Reference Ingalls, Shah, Hansman, Aluwihare, Santos, Druffel and Pearson2006). Steroids and pigments and their derivatives, biomineral occluded compounds and as nucleic acids (Cherrier et al. Reference Cherrier, Bauer, Druffel, Coffin and Chanton1999) have been investigated. Recent developments use CSRA of amino acids to study their sources and cycling (Bour et al. Reference Bour, Walker, Broek and McCarthy2016; Blattmann et al. Reference Blattmann, Montluçon, Haghipour, Ishikawa and Eglinton2020).
All applications of CSRA on marine organic compounds require extraction and purification of sufficient mass of the compound from samples where they are typically present in trace amounts. As a consequence, most CSRA studies are conducted using sediments that have sufficient material available. In most cases, CSRA of compounds isolated from POC or DOC sampled from the water column is not possible, unless dedicated sampling campaigns that collect several thousands of liters of water are conducted (e.g., Ingalls et al. Reference Ingalls, Shah, Hansman, Aluwihare, Santos, Druffel and Pearson2006). An exception was sugars collected from HMW-DOC in surface waters of the North Pacific that had post-bomb Δ14C values (Repeta and Aluwihare Reference Repeta and Aluwihare2006).
Commonly used methods for extraction and purification targeting mostly lipid compounds have been summarized by Mollenhauer et al. (Reference Mollenhauer, Kusch, Eglinton and Pearson2019). Often, a sequence of wet-chemical and chromatographic techniques is applied, and typically samples of 100 µg C or less are obtained. The samples need to be combusted prior to 14C analysis and in many cases will be graphitized. AMS analyses of small samples are conducted using size-matched standards and blanks for normalization. For such small samples, accurate determination of the amount and Δ14C value of extraneous C added to the sample during the various steps of sample preparation is of critical importance (Santos et al. Reference Santos, Southon, Drenzek, Ziolkowski, Druffel, Xu, Zhang, Trumbore, Eglinton and Hughen2010). Recently, isotope dilution techniques are increasingly used to obtain reliable estimates of this extraneous C contribution and its 14C content, which can subsequently be used to correct CSRA results (e.g., Haghipour et al. Reference Haghipour, Ausin, Usman, Ishikawa, Wacker, Welte, Ueda and Eglinton2019; Sun et al. Reference Sun2020).
Taking advantage of the elemental-analyzer-accelerator-mass-spectrometer (EA-AMS) setup, recent methodological developments allow for direct injection of CO2 gas produced from combustion of purified compounds into a gas accepting ion source (Haghipour et al. Reference Haghipour, Ausin, Usman, Ishikawa, Wacker, Welte, Ueda and Eglinton2019; Mollenhauer et al. Reference Mollenhauer, Grotheer, Gentz, Bonk and Hefter2021). Purified compounds are transferred into small tin cups, dried and combusted in the EA (Elementar Elemental Analyzer) coupled to the AMS. Thereby, the graphitization step is omitted, and high-precision 14C data can be obtained for samples containing 50 µg C or less. For samples younger than approximately 5000 years, precise 14C analyses can be achieved for samples as small as 10 µg C (Haghipour et al. Reference Haghipour, Ausin, Usman, Ishikawa, Wacker, Welte, Ueda and Eglinton2019).
Ramped Pyrolysis Oxidation (RPO) and 14C
In the mid-2000s, attempts to understand the chronology of Antarctic sediments led John Hayes to develop the Ramped Pyrolysis Oxidation system (RPO) at the National Ocean Sciences AMS facility, a system that separates organic matter based on thermochemical properties. RPO 14C analysis involves the controlled step heating of samples containing organic C, with or without oxygen, typically at a heating rate of 5°C/minute (Figure 6). As heating proceeds, the most reactive organic components become volatile and are swept into an oxidizing reactor by a helium stream, allowing separation of CO2 from other pyrolysis/combustion components and collection of CO2 over discrete temperature intervals for analysis of both stable and radiocarbon isotopes. A major advantage is the ability to analyze all of the organic matter in a sample, not just an isolated fraction. In sediments, the thermal separation allows the isolation of fresh detrital matter from eroded bedrock. The initial goal of improving the chronologies of Antarctic sediments was realized (Rosenheim et al. Reference Rosenheim, Day, Domack, Schrum, Benthien and Hayes2008) and has been expanded upon (Rosenheim et al. Reference Rosenheim, Santoro, Gunter and Domack2013; Subt et al. Reference Subt, Fangman, Wellner and Rosenheim2016).
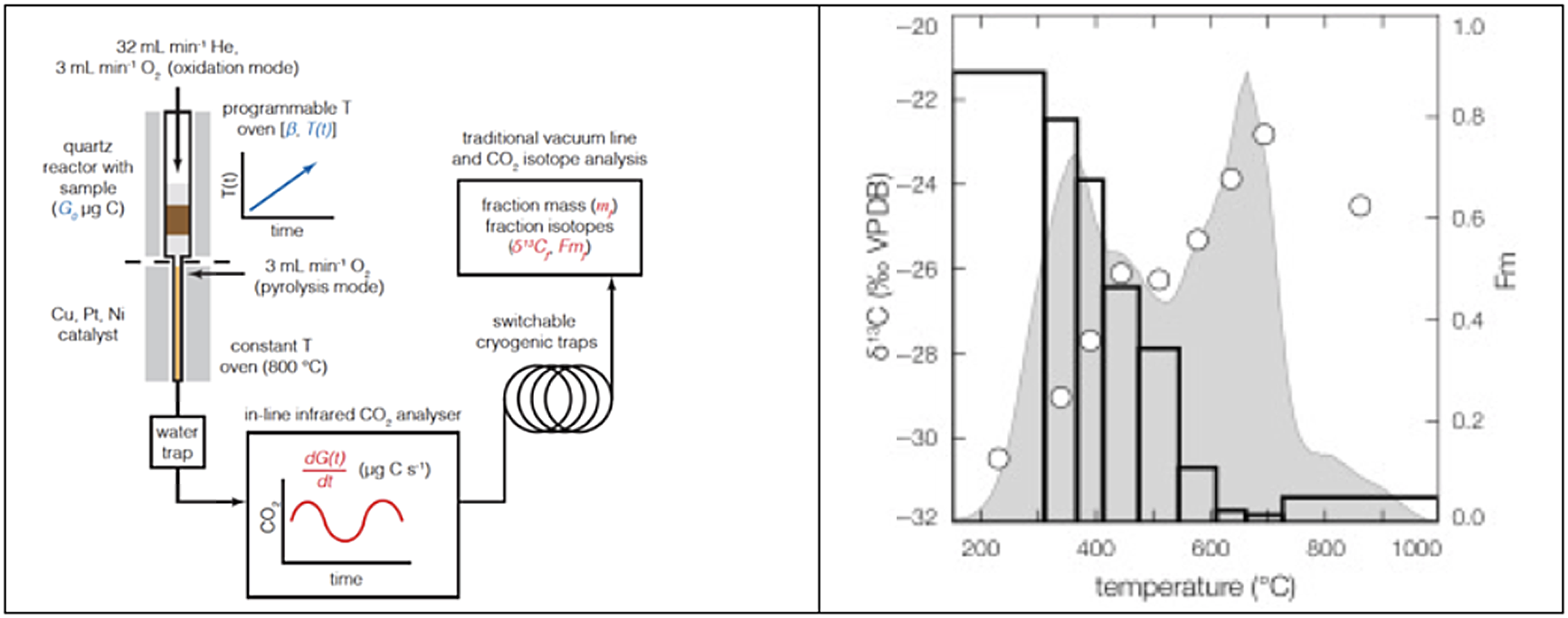
Figure 6 Schematic of the RPO reactor (left) and the results (right) from a POC sample collected from the Narayani River, Nepal (Hemingway Reference Hemingway, Galy, Gagnon, Grant, Rosengard, Soulet, Zigha and McNichol2017). The top half of the quartz reactor heats the sample while the gases are swept into a CO2 analyzer before passing through a cryogenic trap. The gray shaded region is the thermogram, the variation in ppm CO2 vs. temperature (units not shown), white circles show the δ13C values, and bars show the fraction modern values for each temperature interval (Hemingway Reference Hemingway, Galy, Gagnon, Grant, Rosengard, Soulet, Zigha and McNichol2017). The data show that the high temperature, more refractory, fractions have dramatically less 14C, i.e., are older than the lower temperature fractions.

Figure 7 Preliminary F14C POCsusp values of samples collected along a shelf to open ocean transect off Cape Blanc (NW Africa, ∼21°N) collected in November 2018 during research cruise MSM79 onboard the German R/V Maria S. Merian and processed according to the EA–AMS method described above (H. Grotheer unpublished data). Red dots indicate sampling depths. (The map was generated using Ocean Data View; Schlitzer Reference Schlitzer2015).
Building on this success, RPO has since been applied to a wide variety of biogeochemical questions in many environments including the oceans. It is being used to study the nature of the organic material transported to the oceans from rivers (Hemingway et al. Reference Hemingway, Rothman, Grant, Rosengard, Eglinton, Derry and Galy2019). Hemingway et al. (Reference Hemingway, Rothman, Rosengard and Galy2017) developed an inverse method to calculate organic C reactivity from RPO results. They used the method on RPO results from 62 sediment organic C, riverine POC, soil organic C, and DOC samples to indicate that mineral interactions are more important for the preservation of organic C than selective preservation (Hemingway et al. Reference Hemingway, Rothman, Grant, Rosengard, Eglinton, Derry and Galy2019). RPO has been applied to studying the fate of petroleum hydrocarbons, both natural and pollutant, in marine and coastal ecosystems (Pendergraft et al. Reference Pendergraft, Dincer, Sericano, Wade, Kolasinski and Rosenheim2013; Pendergraft and Rosenheim Reference Pendergraft and Rosenheim2014; Adikhari et al. Reference Adhikari, Maiti, Overton, Rosenheim and Marx2016; Rogers et al. Reference Rogers, Bosman, Lardie-Gaylord, McNichol, Rosenheim and Montoya2019). A time series study of the impact of the Deep Water Horizon oil spill on sediments from four sites demonstrated the range of impacts and the time evolution of the oil spill. RPO and C isotope analysis allowed the separation of the spilled petrocarbon from natural hydrocarbon seep C. Emerging work is using the technique to explore the nature of DOC, particularly the high molecular weight fraction.
EA-AMS for Suspended POC 14C
During oceanographic expeditions, suspended POC (POCsusp) for radiocarbon analysis is typically collected using in-situ water pumps with GF/F filters that are lowered to water depths where up to 1000 L are pumped (Griffith et al. Reference Griffith, McNichol, Xu, McLaughlin, Macdonald, Brown and Eglinton2012). Because water pumps are expensive, multiple casts per station are required to obtain full-depth water column 14C POCsusp profiles, a time consuming process that is also expensive.
Utilizing the EA-AMS setup can overcome in-situ water pump limitations, because the MICADAS (MIniCArbonDAtingSystem) allows routine, high precision analysis of small samples (> 100 µgC). For EA-AMS, seawater is processed onboard directly from CTD-ROS (Conductivity Temperature Depth-Rosette) niskin bottles, where 2–40 L of water are collected to obtain sufficient C on GF/F filters, depending on the POCsusp concentration. It is possible to sample up to 24 depths during one deployment and generate high resolution depth profiles of POCsusp 14C (Figure 7). POCsusp is concentrated on pre-combusted GF/F filters using a vacuum supported glass filtration device that allows the sampling of filtrate to obtain the corresponding DOC fraction. In the lab, the filters are acidified, packed into tin capsules, and combusted in the EA. The generated CO2 is measured in the MICADAS via direct injection into the gas ion source (Mollenhauer et al. Reference Mollenhauer, Grotheer, Gentz, Bonk and Hefter2021).
The conventional CuO oxidation reactor of the EA operating at 950°C is prone to cracking, due to halogen radicals reacting with CuO that attack the quartz reactor. Recently, a modified EA reactor has replaced the CuO with WO3 and changed the position of the Ag wool. The WO3 does not react with halogen radicals and Ag wool is positioned at low reaction temperatures further preventing unwanted side reactions. The reaction temperature is lowered to 850°C, and an Inconel liner and ash fingers are used to further protect the quartz reactor. This reactor setup withstands 150–200 samples, whereas a typical CuO reactor processing saline POCsusp filters cannot withstand more than 50 samples.
The disadvantage of this setup is that the C content of the blank increases significantly. The C content of a typical blank for a pre-combusted ½ 45 mm GF/F analyzed on a CuO reactor is ∼10 μg C, but for the modified WO3 reactor it is ∼20 μgC. The higher blank C originates from the WO3 and can be reduced by carefully inspecting the material and removing incompletely oxidized tungsten particles and foreign objects. Nonetheless, pre-combusted GF/F filters contain a significant amount of blank C and appropriate blank determination and subsequent correction is critical. The high blank C content has further implications for the minimum amount of seawater that needs to be filtered per sample in order to acquire an optimum blank C to POCsusp ratio.
In the first example of a high-resolution F14C (Fraction modern 14C) POCsusp profile (Figure 7, H. Grotheer unpublished data) was generated for a transect from the shelf to the open ocean off Cape Blanc (NW Africa, ∼21°N). The Cape Blanc region is located in the southern part of the Canary Current Eastern Boundary Upwelling system off northwest Africa. Here, the Cape Blanc upwelling filament transports cold, nutrient-rich, upwelled waters for hundreds of kilometers offshore towards the oligotrophic ocean (Van Camp et al. Reference Van Camp, Nykjaer, Mittelstaedt and Schlittenhardt1991; Gabric et al. Reference Gabric, Garcia, Van Camp, Nykjaer, Eifler and Schrimpf1993; Àlvarez-Salgado et. al. Reference Àlvarez-Salgado, Doval, Borges, Joint, Frankignoulle, Woodward and Figueiras2001) and is an important transport pathway for particles (Karakas et al. Reference Karakas, Nowald, Blaas, Marchesiello, Frickenhaus and Schlitzer2006).
Fresh POCsusp (F14C 1.0) produced by primary production on the shelf is transported along the continental slope within the bottom layer and travels offshore at ∼500 m water depth. Further offshore, underneath the photic zone, F14C POCsusp decreases to ∼0.8 at 500 m depth. Below 500 m, F14C values show a heterogeneous distribution with pre-aged POCsusp (F14C ∼0.6) separated by layers of POCsusp with intermediate F14C values, suggesting the presence of a refractory POCsusp pool, potentially originating from sediment resuspension. These preliminary data highlight the potential of the EA-AMS method to generate high-resolution, F14C POCsusp profiles that will advance our understanding of POC cycling, transport and sequestration processes in the ocean.
FUTURE WORK
Studies of marine organic C have benefitted from research conducted outside of the marine scientific community. Effort to continue this strong communication and collaboration is important to maintain and to nurture. New ways to unlock the secrets of the biogeochemistry of the marine system will benefit humanity as we chart a course through the Anthropocene.