Fe deficiency is common globally and some subgroups of the population are at particular risk( Reference Zimmermann and Hurrell 1 ). In pregnancy, circulating Fe levels drop owing to the increasing fetal Fe requirements as the pregnancy progresses. This often leads to Fe deficiency, which increases the risk of preterm delivery, low birth weight and neonatal mortality( Reference Coad and Conlon 2 ). Fe deficiency also affects the composition of the gut microbiota and can lead to decreases in bacterial species (including Roseburia spp.) known to produce SCFA( Reference Dostal, Chassard and Hilty 3 ), which can act as signalling molecules. Supplementation is a common strategy to reverse Fe deficiency; however, the proportion of Fe absorbed from oral supplements is often low( Reference Cook and Reddy 4 ). Thus, non-absorbed Fe may reach the colon where it has the potential to alter the composition of the gut microbiota, as Fe is an important determinant of bacterial growth( Reference Prentice, Mendoza and Pereira 5 ).
Fe supplementation in both humans (20 mg/d) and in animal models (10–20 mg/kg diet in rats, 0–250 mg/kg diet in piglets) has had detrimental effects on the composition of the gut microbiota( Reference Dostal, Chassard and Hilty 3 , Reference Lee, Shinde and Choi 6 , Reference Zimmermann, Chassard and Rohner 7 ). Most human supplementation studies have been performed in malnourished anaemic children in low- and middle-income countries. Fe supplementation in these populations has led to decreased abundance of Lactobacilli and increased abundance of Enterobacteriaceae and Bacteroides ( Reference Zimmermann, Chassard and Rohner 7 , Reference Krebs, Sherlock and Westcott 8 ). These changes have been accompanied by increases in calprotectin levels, a marker of gut inflammation( Reference Zimmermann, Chassard and Rohner 7 ). Consistent with this, Fe -supplementation-associated microbiota changes are associated with increased severity of histological colitis( Reference Dostal, Lacroix and Pham 9 ). The changes are also associated with increased frequency and severity of diarrhoeal disease( Reference Lee, Shinde and Choi 6 ), which is associated with mortality( Reference Kotloff 10 ).
In contrast to the findings in low- and middle-income countries, in developed countries, Fe supplementation in children and adults demonstrates different changes in the microbiota, with decreases reported in members of the Coriobacteriales and Clostridiales orders and increases in Bifidobacterium ( Reference Lee, Clavel and Smirnov 11 ). These changes do not result in diarrhoea or worsening of inflammatory bowel disease. Some gut bacteria produce phosphatidylglycerol, palmitate and palmitate derivatives, and serum levels of these are increased in individuals receiving oral supplementary Fe compared with those supplemented intravenously( Reference Lee, Clavel and Smirnov 11 ). A recent study in mice showed that a diet high in Fe increased the abundance of some members of the Clostriadiales and Bacteroidales orders and decreased the abundance of members of the Lactobacillales order( Reference Constante, Fragoso and Lupien-Meilleur 12 ). However, the microbiota composition was differentially affected by the exact formulation of the Fe. At a consistent Fe dose of 50 mg/kg, relative to ferrous sulphate, ferrous bisglycinate increased the abundance of members of the Bacteroidales order and decreased the abundance of Clostridiales and Bacillales orders, whereas ferric EDTA increased the abundance of some members of the Lactobacillales order but decreased the abundance of other members, as well as of members of the Clostridiales order( Reference Constante, Fragoso and Lupien-Meilleur 12 ). The predicted functions of the microbiota with the different supplements also varied. Gut microbiota composition changes throughout pregnancy( Reference Koren, Goodrich and Cullender 13 ) and has been associated with both metabolic( Reference Gomez-Arango, Barrett and McIntyre 14 ) and cardiovascular( Reference Gomez-Arango, Barrett and McIntyre 15 ) markers in the mother, as well as dietary fibre and fat intake( Reference Röytiö, Mokkala and Vahlberg 16 ). Dietary Fe intake is positively correlated with the low-grade inflammatory marker GlycA( Reference Röytiö, Mokkala and Vahlberg 16 ). It is possible that Fe supplementation affects maternal health by altering the composition of the maternal gut microbiota and thereby may affect fetal development. Routine Fe supplementation is not recommended for pregnant women by the Royal Australian and New Zealand College of Obstetricians and Gynaecologists. The college recommends Hb level measurements at the first antenatal visit and at 28 weeks’ gestation and supplementation with ≥60 mg/d if anaemia is diagnosed. However, many pregnancy multivitamin supplements contain Fe at varying doses.
Given the high rates of supplementation with multivitamins in early pregnancy, this study aimed to investigate whether the dose of Fe supplementation affected the composition of the gut microbiota at baseline in a cohort of overweight and obese pregnant women participating in a randomised controlled trial of probiotics to prevent gestational diabetes mellitus (SPRING cohort).
Methods
Study participants and clinical samples
Overweight and obese pregnant women participating in the Study of Probiotics IN Gestational diabetes (SPRING, ANZCTR12611001208998)( Reference Nitert, Barrett and Foxcroft 17 ) who provided a faecal sample were included in this study. All participants provided informed written consent, and the study was approved by the human research ethics committees at the Royal Brisbane and Women’s Hospital (RBW/11/467) and The University of Queensland (2012000080). SPRING is a multicentre randomised clinical trial of probiotics to prevent gestational diabetes mellitus in overweight and obese women. Faecal samples were obtained at baseline (16 weeks’ gestation) before randomisation and the start of the probiotics intervention. At the baseline visit, women also provided a fasting blood sample and clinical information, and completed a detailed FFQ of their nutritional intake during pregnancy (Cancer Council Victoria’s Dietary Questionnaire for Epidemiological Studies DQES version 2.0)( Reference Giles 18 ). Use of pregnancy multivitamins and other food supplements was recorded, including brand and dose if known to the women. All brands of pregnancy multivitamins used contained ferrous Fe. Women were grouped into a low supplementary Fe intake (LI) group with 0–10 mg Fe /d (n 94) or a high supplementary Fe intake (HI) group with ≥60 mg Fe /d (n 65). The grouping reflects the Fe content of pregnancy multivitamins available in Australia, which contain either ≤10 mg of Fe or ≥60 mg of Fe. The HI group includes women taking specific Fe supplements exceeding 60 mg/d (n 11) and those taking multivitamins containing 60 mg/d Fe (n 54). Women whose supplementary Fe intake was uncertain were excluded from this study (see online Supplementary Fig. S1).
DNA isolation
Faecal samples were collected by the participants at home, refrigerated and stored at −80°C within 24 h before the isolation of DNA from 250 mg of sample using the R+BBC method followed by the Qiagen AllPrep DNA extraction kit( Reference Gomez-Arango, Barrett and McIntyre 19 , Reference Yu and Morrison 20 ). Specifically, the sample was mechanically lysed by homogenisation at 30 Hz for 3 min with 0·4 g of a mixture of 0·1 and 0·5 mm sterile zirconia beads. DNA quantity and quality was ascertained by spectrophotometry (Nanodrop ND 1000).
Gut microbiota composition
16S rRNA sequencing
The composition of the gut microbiota in faecal samples was assessed by amplification of the V6–V8 region of the 16S rRNA gene using the barcoded primer set 926F (5'-TCG TCG GCA GCG TCA GAT GTG TAT AAG AGA CAG AAA CTY AAA KGA ATT GRC GG-3') and 1392R (5'-GTC TCG TGG GCT CGG AGA TGT GTA TAA GAG ACA GAC GGG CGG TGW GTR C-3'). DNA isolated from Escherichia coli JM109 was used as positive control and deionised sterile water as a negative control in each PCR run. The Nextera XT V2 index kit Set A and B was used for barcoding the PCR products, and were purified with the AMPure XP bead system. Barcoded DNA was quantified, normalised and pooled before sequencing on the Illumina MiSeq platform at the University of Queensland’s Australian Centre for Ecogenomics (ACE). The Quantitative Insights Into Microbial Ecology (QIIME) version 1.9.1 analysis pipeline( Reference Caporaso, Kuczynski and Stombaugh 21 ) was used to join, de-multiplex and quality filter the generated forward and reverse sequences. Operational taxonomic units (OTU) were identified using the open reference OTU picking method with the Greengenes reference database using a pairwise identity threshold of 97 %. OTU detected in the negative controls or with an abundance of <0·0001 were removed from the OTU table. The OTU table was rarefied to 3000 sequences/sample before performing downstream analysis; all samples had ≥3000 reads.
Statistical analysis
As the 16S rRNA data were not normally distributed, they are presented as medians and interquartile ranges. Non-parametric statistical methodology (Mann–Whitney U tests, Spearman’s rank correlation coefficient testing) was used and a P value of<0·05 was considered statistically significant. The QIIME and Calypso ((http://bioinfo.qimr.edu.au/calypso/) version 7.12( Reference Zakrzewski, Proietti and Ellis 22 ) software tools were used for analysis of the gut microbiota composition. Alpha diversity of the gut microbiota was determined by the Chao-1 and Shannon indices. Principal component analysis was used to test for beta diversity using the Adonis statistical test for permutation multivariate ANOVA. Network analysis was performed to identify co-occurring bacteria and mutually exclusive bacteria in the LI and HI groups. Taxa were associated with supplementary Fe intake using Spearman’s rho and Bray–Curtis dissimilarity distances with 1000-fold permutations. The results are reported as significant if the false discovery rate (FDR) was<0·05, with the degree of colouring of the nodes reflecting the level of significance of the association with supplemental Fe intake group. The predicted functions of the microbiome were analysed with the PICRUSt software tool. LefSe (linear discriminant analysis effect size) analysis was carried out to identify functional pathways discriminating between the groups.
Justification of sample size
In this analysis, sample size was determined by the availability of a faecal sample and information about supplemental Fe intake. However, the group sizes of ninety-four and sixty-five individuals, respectively, enable to distinguish a 30 % change in abundance between the groups for bacteria with a relatively low mean abundance of 1 % of the gut microbiota composition and a sd of 0·8 %.
Results
Participants
Of the 210 participants in the SPRING study with faecal samples, 159 women had detailed supplementary Fe intake information, with ninety-four women having low supplementary Fe intake (LI group) and sixty-five women having high supplementary Fe intake (HI group) (Table 1). The Fe in the supplements taken by the women in the LI group consisted of either ferrous sulphate or ferrous glycinate. In the HI group all the supplemental Fe was as ferrous sulphate. The groups did not differ in maternal age, BMI, gestational age at study entry, systolic or diastolic blood pressure or fasting glucose levels. In addition, dietary Fe intake levels were similar in both groups, about 10 mg/d. Dietary protein intake levels were also similar in both groups and correlated strongly with dietary Fe intake levels (ρ=0·88, P<0·0001).
Table 1 Participant characteristics(Medians and interquartile ranges (IQR) and ranges)

LI, low supplementary Fe intake; HI, high supplementary Fe intake; BP, blood pressure.
Overall gut microbiota composition
Alpha diversity was not different between the groups (results not shown). Beta diversity was significantly different between the groups (R=0·037, P=0·031) (Fig. 1(a)); however, the R-value is close to 0, indicating that the difference was small. Furthermore, there were no major clustering differences in gut microbial composition between the groups at any taxonomic level (genus level presented as Fig. 1(b)). The abundance of bacteria previously noted in the literature to be differentially affected by supplementary Fe intake was analysed (Table 2). The abundance of the genera Roseburia and Ruminococcus was significantly lower in the HI group (P=0·045 and P=0·038), but this was no longer significant after correction for multiple testing (FDR 0·23 and 0·18, respectively). Network analyses showed that the gut microbiota of women with low supplementary Fe intake was dominated at the genus level by bacteria known to contain short-chain fatty acid producers, including Ruminococcus, Suterella and Lachnospira, but also the lactate producer Lactobacillus (Fig. 2). In women with high supplementary Fe intake, only Acidaminococcus was over-represented. However, it should be noted that the differences are small, as indicated by the lack of bright nodes in the network.

Fig. 1 Overall composition of the gut microbiota in women with high or low supplementary iron intake. (a) Beta-diversity plot for supplementary iron intake showing diversity of the gut microbiota within the high-iron supplementation group, within the low supplementation group and between the groups. Beta-diversity was assessed by Anosim and was found to be significantly different between the groups. (b) Principal component analysis (PCA) plot at the genus level for women with high () and low (
) supplementary iron intake.
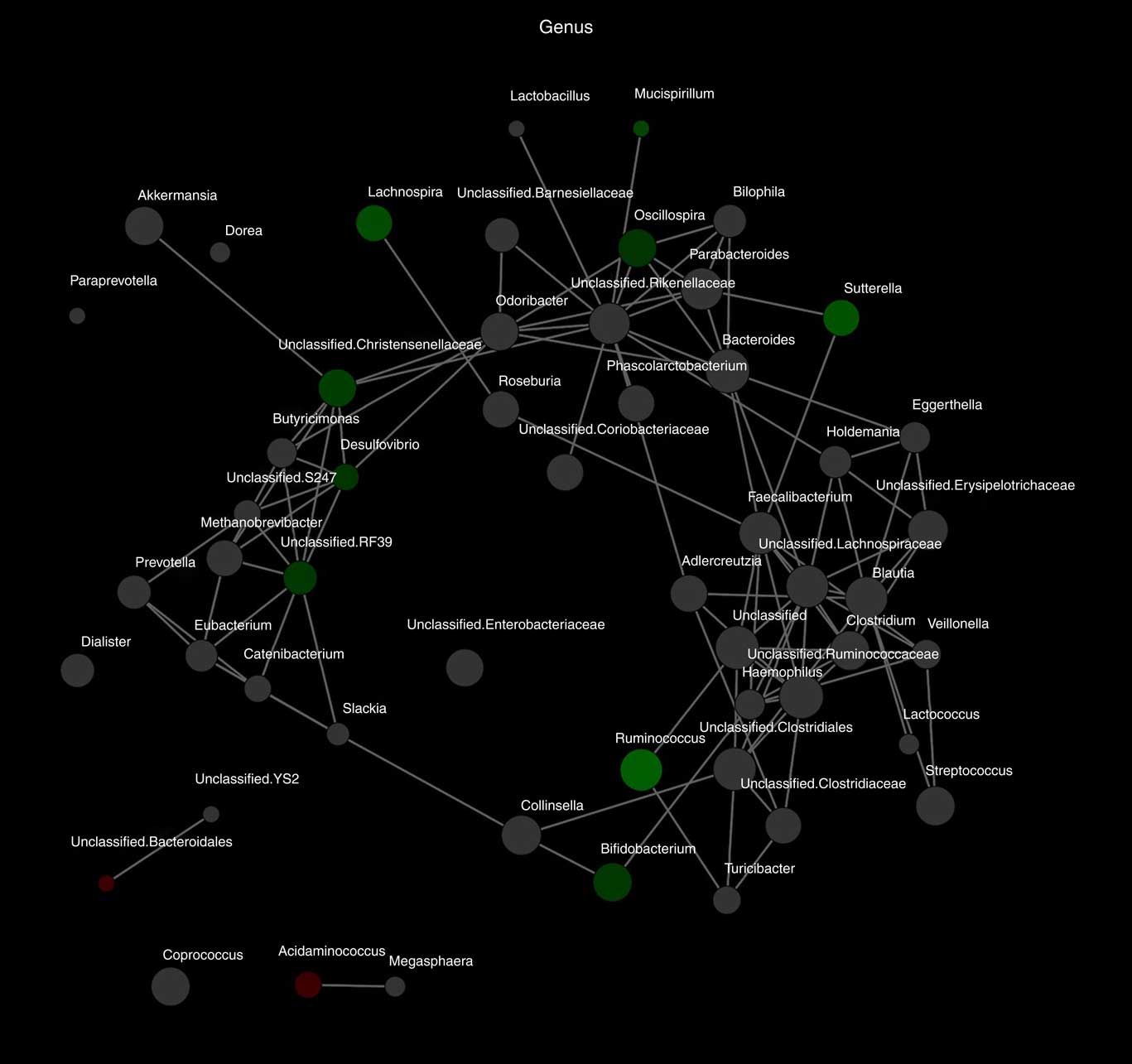
Fig. 2 Differences in microbiota composition according to high and low supplementary iron intake. Network analysis based on high (n 65) and low (n 94) supplementary iron intake. Genera are represented as nodes. Green nodes indicate genera over-represented in the low-iron group. Red nodes indicate genera over-represented in the high-iron group. Colour intensity explains the strength of the associations with supplementary iron intake, with more colour representing a stronger association.
Table 2 Analysis of the abundance of bacteria previously shown to be affected by supplemental iron intake

LI, low supplementary Fe intake; HI, high supplementary Fe intake; FDR, false discovery rate.
Differences in the predicted function of the gut microbiota
The predicted functions of the gut microbiota in the LI and HI groups were compared. The gut microbiota in LI group was associated with more pronounced butanoate metabolism and lipid biosynthesis proteins, whereas in the HI group amino acid metabolism and siderophore biosynthesis were more pronounced (Fig. 3(a) and (b)).

Fig. 3 Predictive functional analysis of metabolism and biosynthesis of the gut microbiota in the low-iron (LI) and high-iron (HI) supplementation groups. (a) Metabolic pathways significantly different in the LI () and HI (
) groups. (b) Significantly different predicted biosynthetic pathways in the LI and HI groups. LDA score, linear discriminant analysis score of predicted pathways discriminating between LI and HI groups.
Discussion
The results of this study show that the routine supplementation with Fe in multivitamin capsules in early pregnancy is not associated with under- or over-abundance of specific genera in the gut microbiota. In the network analysis, there was a slightly higher predominance of bacteria that include known short-chain fatty acid producers in the women who had no/low levels of Fe supplementation. To our knowledge, this is the first study of the effects of Fe supplementation on the composition of the gut microbiota in pregnancy.
Functional predictions of the gut microbiota in women with low supplementary Fe indicated that butanoate (butyrate) metabolism was increased. Higher faecal butyrate concentrations have been associated with increased abundance of the genus Roseburia from the family Lachnospiraceae( Reference Barton, Penney and Cronin 23 ). In our study, Roseburia abundance tended to be higher in the gut microbiota of women with low Fe supplementation . These findings are consistent with rodent studies that reported that Fe supplementation reduced the abundance of Roseburia spp. in Fe -deficient animals( Reference Dostal, Lacroix and Pham 9 ). In humans, Fe supplementation has also been reported to decrease the abundance of Roseburia ( Reference Constante, Fragoso and Lupien-Meilleur 12 ). Similarly, the genus Ruminococcus in the family Ruminococcaceae is also a known butyrate producer and trended towards lower abundance in the gut microbiota of women with high Fe supplementation( Reference Zhang and Davies 24 ). In the gut, increased butyrate metabolism may contribute to a stronger intestinal barrier, a thicker mucus layer and reduction of pro-inflammatory cytokines( Reference van der Beek, Dejong and Troost 25 ). Given the changes noted with Fe supplementation, the decreases in SCFA producers, which mainly synthesise acetate, propionate and butyrate, may alter gut barrier function. Butyrate metabolism is decreased in the gut microbiota of patients with known gut barrier function disorders such as Crohn’s disease( Reference Wright, Kamm and Teo 26 ). Decreased butyrate metabolism has previously been described in the placental microbiota of women with excess gestational weight gain who delivered prematurely( Reference Antony, Ma and Mitchell 27 ). In pregnant women, impaired intestinal barrier function could increase the inflammatory state of the mother, which could have an impact on the size of the baby. However, there was no difference in infant birth size in our cohort. Our findings with Fe supplementation need to be investigated further to explore the relationships between Fe supplementation and other obstetric outcomes.
The network analysis in women with higher supplemental Fe intake showed a microbiota with a higher abundance of Acidaminococcus. Acidaminococcus, a member of the Firmicutes phylum, has been associated with changes in glucose metabolism and obesity. In patients undergoing bariatric surgery, the abundance of Acidaminococcus increases; however, this was not correlated with any metabolic markers including fasting serum glucose, insulin or cholesterol levels in the patients( Reference Patrone, Vajana and Minuti 28 ). Acidaminococcus spp. are known amino-acid-fermenting bacteria, and exclusively metabolise glutamate for carbon and energy supply. It has been hypothesised that the increase in undigested protein after bariatric surgery could potentially explain the increased abundance of Acidaminococcus ( Reference Patrone, Vajana and Minuti 28 ). However, in our cohort, dietary protein intake was similar between the groups, and it is likely that, in healthy pregnant women, the majority of the protein is digested before entering the colon. Undigested protein is therefore unlikely to explain the difference in Acidaminococcus in our study.
Low protein intake and competition for glutamate between the host gut epithelium and Acidaminococcus may negatively affect gut barrier function and growth in children, potentially by affecting nitrogen metabolism and urea recycling in the gut lumen( Reference Gough, Stephens and Moodie 29 ). In undernourished children, Acidaminococcus abundance is higher in children with stunting and negatively correlated with height-over-age z scores( Reference Gough, Stephens and Moodie 29 ). Acidaminococcus can be detected in children with newly diagnosed type 1 diabetes, but not in their matched normoglycaemic counterparts( Reference Qi, Zhang and Yu 30 ). Type 1 diabetes has also been associated with increased gut leakiness( Reference Li and Atkinson 31 ), providing a common mechanism for the gut microbiota to affect the health of the host.
In our study, the microbiota of women receiving high Fe supplementation had higher predicted siderophore biosynthesis and amino acid metabolism. This was an unexpected finding. Siderophores are low-molecular-weight Fe carriers and serve to bind Fe, especially Fe3+, with high affinity( Reference Neilands 32 ). They are usually produced by bacteria and fungi in response to low-Fe environments( Reference Neilands 32 ), which is opposite to our HI group. Siderophores comprise multiple different compounds, which can have distinctive functions and affinities for ferric and ferrous Fe, and bacteria may use a composite of different siderophores to ensure survival( Reference Ellermann and Arthur 33 ). Our predictive functional analysis does not distinguish between different forms of siderophores. The predominance of siderophore biosynthesis in the HI group may reflect a microbiota where bacteria capable of producing siderophores are over-represented, as some bacteria, including certain Lactobacillus spp., do not synthesise siderophores( Reference Neilands 32 ). Most supplements contain ferrous Fe (Fe2+), which has been shown to down-regulate the synthesis of siderophores in Fe -replete states, possibly to prevent oxidative stress( Reference Touati, Jacques and Tardat 34 ). It is possible that, although the microbiota of women receiving high Fe supplementation contains more bacterial species that produce siderophores, the actual rates of synthesis are unchanged. We do not know the Fe status or the duration of Fe supplement use in our cohort, and thus it is possible that women with a history or presence of Fe deficiency had chosen to commence the higher-Fe -containing Fe supplement. The conditions and bacteria required for actual bacterial siderophore production in the human intestine are still largely unknown( Reference Ellermann and Arthur 33 ), and our findings need to be confirmed in other data sets.
In this cohort of women, we were not able to definitively determine their Fe status. However, although the HI group may include some women with low Fe status who were prescribed Fe supplementation by their health care provider, the majority of this group (83 %) used a multivitamin-containing 60 mg/capsule of Fe as indicated by the median and range displayed in Table 1. It is likely that the study population resembles the general obstetric population in Fe status and multivitamin use in Australia( Reference Shand, Walls and Chatterjee 35 ), where up to 97·5 % use multivitamins throughout the first trimester. All multivitamin supplements used by the women in this study contained ferrous Fe, with the high-Fe supplement consisting solely of ferrous sulphate, and the women in the LI group were taking a supplement containing either ferrous sulphate or ferrous glycinate. There was no indication that the form of Fe altered the gut microbiota. The fact that there were no specific genera of bacteria that were altered in women with high Fe supplementation suggests that this level of supplementation does not markedly alter the composition and thereby the function of the gut microbiota. Changes to the gut microbiota in response to supplementation or dietary changes are generally rapid( Reference David, Maurice and Carmody 36 ), and it is therefore unlikely that the lack of differences observed is due to the duration of supplementation. Given that this cohort only included overweight and obese women, the impact of multivitamin supplementation incorporating Fe on the gut microbiota in lean women needs to be examined. However, the fact that there were no differences in BMI between the groups in this study suggests that our findings are independent of BMI. Furthermore, whether or not maternal Fe status is associated with changes in the gut microbiota of pregnant women and whether Fe supplementation rectifies any alterations in women with low Fe status remain to be determined. Furthermore, recent studies emphasised the effects of dietary fibre, fat and vitamin intake on gut microbiota composition in early pregnancy and early postpartum( Reference Röytiö, Mokkala and Vahlberg 16 , Reference Mandal, Godfrey and McDonald 37 ). It is possible that the differences in dietary fibre and fat intake between individual women mask any differences due to supplementary Fe intake. However, this again suggests that supplementary Fe intake only has a minor effect on gut microbiota composition. Last, this study was powered to observe a difference of ≥30 % in abundance of relatively low-abundant bacteria (mean 1 (sd 0·8) %). The network analyses suggest that smaller changes may be present: if the functional outcome of the small changes is in the same direction, these may be physiologically important. A larger 16S rRNA study or a meta-genomic analysis of the genes present or expressed by the bacteria could clarify this further.
Conclusion
In conclusion, the results of this study suggest that the Fe levels up to 60 mg/d in pregnancy multivitamins do not significantly alter the overall composition of the gut microbiota in early pregnancy. Network analyses indicated that supplementation with lower amounts of Fe is correlated with a slightly higher abundance of SCFA producers, which may be beneficial in maintaining gut barrier integrity.
Acknowledgements
The authors thank the SPRING participants; the SPRING trial group included Katie Foxcroft, Anne Tremellen, Sharney Grant, Dr Jacinta Tobin, Dr Shelley Wilkinson, Dr Chris McSweeney, Prof Peter O’Rourke and Dr Barbara Lingwood.
L. F. G.-A. is supported by a Colciencias scholarship from the government of Colombia. H. L. B. is supported by an National Health & Medical Research Council (NHMRC) Early Career Fellowship (grant no. APP1120070). The SPRING study is supported by the NHMRC (grant no. 1028575), Diabetes Australia Research Programme and the Royal Brisbane and Women’s Hospital Foundation.
M. D. N. designed the research, conducted the study and analysed the data, and wrote and edited the manuscript. L. F. G.-A. researched data and performed analysis. L. F. G.-A., H. L. B., D. M. F., H. D. M., L. K. C. and G. J. A. provided intellectual input and edited the manuscript. H. L. B. and D. M. F. designed the research. H. D. M. and L. K. C. provided samples.
The SPRING study has received probiotics and placebo products from Chr Hansen A/S. The authors declare that there are no conflicts of interest.
Supplementary material
For supplementary material/s referred to in this article, please visit https://doi.org/10.1017/S0007114518001149