Implications
Selection for residual feed intake (−RFIfat; efficient) will result in cattle that consume less dry matter intake, have improved feed conversion ratio and reduced enteric CH4 emissions at equal levels of production, body size and body fatness. Rate of genetic change, using multi-trait selection and a comprehensive record keeping system, is expected to be 0.75% to 1.0% per year compared with no selection for RFIfat. There will be few, if any, antagonistic effects on growth, carcass quality, fertility and cow lifetime productivity. Direct selection for an enteric CH4 phenotype must be viewed with caution since enteric methane has low to moderate repeatability (0.16 to 0.55) with distinctive diurnal patterns associated with cattle type, diet and genotype, necessitating whole day measurement for at least 35 days. Furthermore, the relationships of enteric CH4 production with other economically important traits are largely unknown.
Introduction
Methane (CH4) is the major greenhouse gas (GHG) emitted from ruminant production systems with CH4 from enteric fermentation accounting for 12% to 17% of GHG emissions (Beauchemin et al., Reference Beauchemin, McAllister and McGinn2009). In North America, the cow herd is responsible for about 85% of GHG emissions, where 6 to 7 month old weaned calves are adjusted to a high grain diet over 1 to 1.5 months, fed for 6 months and then harvested at 14 to 16 months of age (Beauchemin et al., Reference Beauchemin, Janzen, Little, McAllister and McGinn2010; Basarab et al., Reference Basarab, Baron, López-Campos, Aalhus, Haugen-Kozyra and Okine2012a). In a calf-to-beef system that couples backgrounding and finishing, more CH4 is emitted from the forage-fed backgrounded phase than from the grain-based finishing phase and the cow herd is responsible for almost 70% of GHG emissions. The high proportion of CH4 associated with the cow herd is because of the cow herd consuming a higher proportion of the feed in a calf-to-beef system, inherently low biological efficiency of the beef cow and the very high proportion of the cow herd's diet as conserved forage, pasture, range and crop residues rather than concentrates (Allen et al., Reference Allen, Fontenot, Notter and Hammes1992; Verge et al., Reference Verge, Dyer, Desjardins and Worth2008; Capper, Reference Capper2011). The impact of roughage v. concentrate diets on increasing the CH4 emissions in ruminant systems is well known (Johnson and Johnson, Reference Johnson and Johnson1995; Beauchemin and McGinn, Reference Beauchemin and McGinn2005; Beauchemin et al., Reference Beauchemin, Kreuzer, O'Mara and McAllister2008). Reducing daily CH4 emissions by increasing cereal grain content in backgrounding and finishing diets and increasing the starch content of small grain and corn silages can be an effective mitigation method that fits logically into the feedlot system (Beauchemin and McGinn, Reference Beauchemin and McGinn2005; Beauchemin et al., Reference Beauchemin, McAllister and McGinn2009).
Methane mitigation for the cow herd may be achieved by improving the digestibility of annual and perennial forages through grazing management, increasing legume composition and utilization of species containing secondary metabolites such as tannins or saponins that affect methanogenesis in the rumen (Beauchemin et al., Reference Beauchemin, Kreuzer, O'Mara and McAllister2008 and Reference Beauchemin, McAllister and McGinn2009). Use of high-sugar perennial ryegrass (Lolium multiflorum L.) cultivars and increasing alfalfa (Medicago sativa L.) and clover (Trifolium repens L.) sward content, where practical, can help improve forage digestibility, although increasing long-term legume content of swards can prove difficult (Dewhurst et al., Reference Dewhurst, Delaby, Moloney, Boland and Lewis2009; Clark et al., Reference Clark, Kelliher and Pinares-Patiño2011) . However, many tannin containing species showing potential are weak competitors with grasses and have a narrow climatic and geographic adaptation, necessitating a long-term plant breeding process (Abberton et al., Reference Abberton, MacDuff, Athole and Humphreys2007) to improve agronomic traits such as winter hardiness, seedling vigor and competitive capacity. Thus, widespread renovation of grasslands to incorporate tannin and saponin-containing species to reduce CH4 production may not be practical.
Reducing cow herd CH4 emission is a necessity for improving the carbon footprint of beef production which ranges from as low as 17 kg carbon dioxide equivalents (CO2e)/kg carcass beef for feedlot finished beef in Canada (Verge et al., Reference Verge, Dyer, Desjardins and Worth2008; Beauchemin et al., Reference Beauchemin, Janzen, Little, McAllister and McGinn2010; Basarab et al., Reference Basarab, Baron, López-Campos, Aalhus, Haugen-Kozyra and Okine2012a) to as high as 40 CO2e/kg carcass beef for grass-finished beef in Brazil (Cederberg et al., Reference Cederberg, Persson, Neovius, Molander and Clift2011). Ultimately, the combined impacts of plant and livestock breeding for low CH4 emission rates may result in substantial reductions of enteric CH4. However, the most practical and rapid mitigation procedure may be to reduce the per cow CH4 emission through animal breeding and genetic selection for feed efficiency as it is permanent and cumulative (Alford et al., Reference Alford, Hegarty, Parnell, Cacho, Herd and Griffith2006). It is also easier to improve efficiency within the cattle population as opposed to the riskier re-establishment or renovation of long-term grasslands, much of which exists on marginal and often arid lands. Furthermore, as the global population surpasses nine billion by 2050, improving feed efficiency becomes even more urgent to meet the increasing demand for feed grains for food and ethanol production (FAO, 2009). Moreover, feeding more grain to the cow herd is not a sustainable practice and feeding additives and supplements on pasture is not practical.
Thus, the purpose of this paper is to discuss genetic selection for feed efficiency, specifically RFI, as an indirect approach to reducing CH4 emissions in ruminants. The impact of RFI on economically important traits will be discussed, as well as the impact of within- and between-animal variation in feed intake on CH4 emissions.
Feed efficiency and RFI
Feed is the largest variable cost and an important determinant of profitability in beef production (ARD, 2005; Ramsey et al., Reference Ramsey, Doye, Ward, McGrann, Falconer and Bevers2005). In North America, 55% to 75% of the total costs of calf-to-beef production systems are associated with feed costs (NRC, 2000; ARD, 2005). The cattle herd (cows, bulls and breeding replacements) consumes 82% of the feed inputs in a calf-to-beef systems where animals are harvested at 11 to 14 months of age and 63% to 64% of the feed inputs in a calf-to-beef system where animals are harvested at 19 to 23 months of age (Basarab et al., Reference Basarab, Baron, López-Campos, Aalhus, Haugen-Kozyra and Okine2012a). In Irish grass-based calf-to-weanling and calf-to-beef systems the cow herd consumes about 85% and 50% of the total feed inputs, respectively (McGee, Reference McGee2009). About two-thirds of the feed energy is required for body maintenance (Ferrell and Jenkins, Reference Ferrell and Jenkins1985; Montano-Bermudez et al., Reference Montano-Bermudez, Nielson and Deutscher1990), and considerable animal-to-animal variation, independent of body size and growth, exists in maintenance requirements of cattle (Herd and Bishop, Reference Herd and Bishop2000; Arthur et al., Reference Arthur, Renand and Krauss2001a and Reference Arthur, Archer, Johnson, Herd, Richardson and Parnell2001b; Basarab et al., Reference Basarab, Price, Aalhus, Okine, Snelling and Lyle2003; Nkrumah et al., Reference Nkrumah, Okine, Mathison, Schnid, Li, Basarab, Price, Wang and Moore2006; Crowley et al., Reference Crowley, McGee, Kenny, Crews, Evans and Berry2010). Thus, improving feed efficiency through genetic selection holds significant opportunity for the beef industry. Traditionally, feed efficiency in beef cattle was defined as feed to gain ratio or FCR. However, genetic evaluation of ratio traits like FCR or methane yield (g CH4/kg dry matter intake (DMI)) are problematic in that selection response is unpredictable, usually placing higher than expected emphasis on the trait with higher genetic variance (Gunsett, Reference Gunsett1984; Kennedy et al., Reference Kennedy, Van der Werf and Meuwissen1993; van der Werf, Reference Van der Werf2004). Further, the genetic correlation between the numerator (e.g. DMI) and denominator (e.g. average daily gain (ADG)) is positive (Koots et al., Reference Koots, Gibson, Smith and Wilton1999; Berry, Reference Berry2012), and therefore, selection for improved FCR has resulted in cattle that grow faster, have increased mature size, and increased maintenance and feed requirements (Bishop et al., Reference Bishop, Davis, Harvey, Wilson and VanStavern1991; Archer et al., Reference Archer, Richardson, Herd and Arthur1999; Herd and Bishop, Reference Herd and Bishop2000; Crews, Reference Crews2005; Kelly et al., Reference Kelly, McGee, Crews, Fahey, Wylie and Kenny2010a and Reference Kelly, McGee, Crews, Sweeney, Boland and Kenny2010b). As a result, efficiency measures that remove various known energy uses from feed intake, such as BW and production, are being used within breeding programs. RFI, also referred to as net feed efficiency, was first proposed by Koch et al. (Reference Koch, Swiger, Chambers and Gregory1963) and was defined as the difference between an animal's actual feed intake and its expected feed requirement for maintenance of body size and production. Low RFI in growing animals represents individuals with lower feed intake at equal body size and growth, with lower maintenance energy requirements and thus greater efficiency. More recently, RFI values have been adjusted for body fatness (RFIfat), thus attempting to render RFI independent of carcass fatness in slaughter cattle and later maturity or fattening in replacement heifers and bulls (Basarab et al., 2003, Reference Basarab, McCartney, Okine and Baron2007 and 2011; Schenkel et al., Reference Schenkel, Miller and Wilton2004; Crews, Reference Crews2005). Similarly, residual gain (RG) is adjusted for body size and DMI and represents animals with superior gain at equal levels of BW and DMI, and a trait that combines both RFI and RG, referred to as residual intake and gain (RFI–RG) represents efficient, fast growing animals that consume less feed (Crowley et al., Reference Crowley, McGee, Kenny, Crews, Evans and Berry2010; Berry and Crowley, Reference Berry and Crowley2012). These latter two measures of feed efficiency should also be adjusted for body composition using final off-test ultrasound backfat thickness, marbling score and/or ribeye area. These measures of feed efficiency are heritable (h 2 = 0.26 to 0.43) and either moderately (RFI v. RG, r g = −0.46, r p = −0.40) or highly (RG v. RFI–RG, r g = −0.87, r p = −0.85) correlated with each other (Crews, Reference Crews2005; Crowley et al., Reference Crowley, McGee, Kenny, Crews, Evans and Berry2010; Berry and Crowley, Reference Berry and Crowley2012). In lactating dairy cows, RFI is defined as the difference between an animal's actual feed intake and its expected feed requirement for BW, fat mobilization, as well as milk fat, protein and yield (Rius et al., Reference Rius, Kittelmann, Macdonald, Waghorn, Janssen and Sikkema2012).
Feed intake, variation and repeatability
All direct measures of feed efficiency require accurate measurement of feed intake and energy uses such as BW, growth and body composition in young cattle (Arthur et al., Reference Arthur, Renand and Krauss2001a and Reference Arthur, Archer, Johnson, Herd, Richardson and Parnell2001b; Basarab et al., 2003, Reference Basarab, McCartney, Okine and Baron2007 and 2011), and BW, fat mobilization and milk fat, protein and yield in lactating dairy cattle (Rius et al., Reference Rius, Kittelmann, Macdonald, Waghorn, Janssen and Sikkema2012). Typically RFI is measured in young cattle (7 to 10 months of age; maximum age difference = 60 days) in feedlot pens fitted with feeding stations designed to automatically monitor individual animal feed intake (e.g. GrowSafe Systems Ltd, Airdrie, Alberta, Canada) following a 21 to 28 days adjustment to their test diet (Basarab et al., 2003 and 2011). The adjustment period is followed by a 76-day test period, which has been recommended as being adequate for the determination of feed intake and growth (Wang et al., Reference Wang, Nkrumah, Li, Basarab, Goonewardene, Okine, Crews and Moore2006). Cattle are weighed before feeding on 2 consecutive days at the start and end of the test period and at ∼14- to 28-day intervals. They are also measured for ultrasound backfat thickness (mm), longissimus thoracis area (cm2) and marbling score at the start (optional) and end of the test period.
Many factors affect the DMI of cattle such as body size, growth, body composition, gender, age, season, ambient temperature, physiological status, previous nutrition and diet (NRC, 2000). Most of these factors are either equal between animals during a standardized feed intake test (e.g. gender, season, ambient temperature and physiological status) or are adjusted for, such as body size, body composition and growth. However, considerable within- and between-animal variation exists in DMI and measures of feed efficiency. The average daily feed intakes and coefficients of variation (CV) for 61 replacement heifers are presented in Figure 1 (J.A. Basarab, 2012; unpublished). The daily CV for feed intake ranged from 11% to 22% and are a reflection of the daily between-animal variation in feed intake. The between-animal repeatability as determined by the ratio-of variance method and conducted in 10-day intervals (e.g. 1 to 10, 1 to 20, 1 to 30 days) ranged from 0.325 to 0.358 for feeder heifers, 0.341 to 0.407 for feeder steers, 0.286 to 0.380 for replacement heifers, 0.374 to 0.449 for young bulls and 0.361 to 0.491 for cows (Table 1). These levels of repeatability are weak to moderate, meaning that an animal was unable to repeat its feed intake consistently over days as reflected by the deceasing repeatability estimates for the same group of cattle as the feeding interval increased (e.g. feeder steers, replacement heifers). Kelly et al. (Reference Kelly, McGee, Crews, Sweeney, Boland and Kenny2010b) reported a similar repeatability of 0.34 for DMI of feeder heifers weighing 418 kg (s.d. = 31.5 kg) fed a total mixed ration consisting of 70 : 30 concentrate and corn silage on a dry matter (DM) basis. Wang et al. (Reference Wang, Nkrumah, Li, Basarab, Goonewardene, Okine, Crews and Moore2006) reported that the phenotypic variances for DMI decreased rapidly from 7 to 35 days of feed intake data collection and then stabilized after 35 days, indicating that extending the duration of data collection beyond 35 days resulted in only small improvement in accuracy.
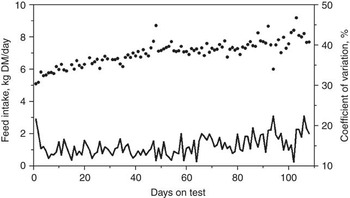
Figure 1 Daily feed intake (solid dots) and coefficient of variation (solid line) averaged for 61 beef heifers (8 to 12 months old) fed ad libitum a 78.2% barley silage and 21.8% barley grain diet (dry matter basis) over 108 days (Basarab, 2012, unpublished).
Table 1 Diet ingredient composition, length of feed intake tests and repeatability of feed intake by period for various beef cattle types (Basarab, 2012, unpublished)
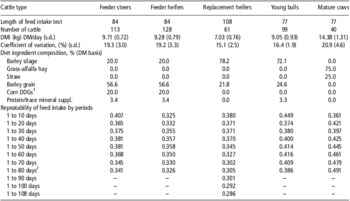
DMI = dry matter intake; DM = dry matter.
1Corn-based dried distillers grains and solubles.
2Feed intake period length was 1 to 84 days for feeder steers and heifers and 1 to 77 days for young bulls and mature cows.
The within-day pattern of feed intake for 61 replacement heifers fed ad libitum a high forage diet is illustrated in Figure 2. The animals were fed once daily at about 0930 h. The repeatability of hourly DMI (00:00:00 to 00:59:59 = 0:00 h, 01:00:00 to 01:59:59 = 1:00 h, …) across 9 days was low for hour 0:00 to 8:00 (r = 0.04 to 0.13), 10:00 to 18:00 (r = 0.02 to 0.16) and 19:00 to 23:00 (r = 0.09 to 0.28), and moderate for hour 09:00 (r = 0.42) indicating considerable within-day within animal variation in feed intake. Thus the within-day repeatability of feed intake is affected when the animal is offered or obtains its feed and varies by RFI phenotype. Collectively, these results suggest that since enteric CH4 production is proportional to DMI (Grainger et al., Reference Grainger, Clarke, McGinn, Auldist, Beauchemin, Hannah, Waghorn, Clark and Eckard2007), within- and between-day enteric CH4 emissions would be at least as variable as daily DMI and would require at least 35 continuous days of recording for a given diet and animal type (e.g. feeder steers on a finishing diet, replacement heifers on a growing diet).

Figure 2 Daily feed intake pattern of the 10 highest and 10 lowest heifers for residual feed intake (RFIfat; 78.2% barley silage and 21.8% barley grain diet, ad libitum, dry matter basis; Basarab, 2012, unpublished).
Repeatability of RFI across diets
Because RFI is a relatively new trait, there are questions regarding its repeatability at different stages of an animal's life, on different diets and in different environments. Moderate-to-high positive phenotypic (r p) and genetic (r g) correlations, and repeatability (r) have been reported between RFI measured on a grower diet and then again on a finisher diet (r g = 0.55, Crews et al., Reference Crews, Shannon, Genswein, Crews, Johnson and Kendrick2003; r p = 0.67, Carstens and Tedeschi, Reference Carstens and Tedeschi2006; r = 0.62, Kelly et al., Reference Kelly, McGee, Crews, Sweeney, Boland and Kenny2010b), and between post-weaning RFI in heifers and when measured again later in life as mature cows (r g = 0.98; Archer et al., Reference Archer, Reverter, Herd, Johnston and Arthur2002). Lower repeatability estimates (r p = 0.33 to 0.42) have been reported by Durunna et al. (Reference Durunna, Mujibi, Goonewardene, Okine, Basarab, Wang and Moore2011) when RFI was measured between two successive feed intake test periods varying in dietary energy content (low v. high energy) and ambient temperature. In their study, 51% to 58% of the animals re-ranked by 0.5 s.d. (0.295 kg DM/day) from the grower phase to the finisher phase which is similar to the 54% identified by Kelly et al. (Reference Kelly, McGee, Crews, Sweeney, Boland and Kenny2010b). This level of re-ranking for RFI, DMI and ADG occurred whether the diet changed from a grower to finisher diet or stayed the same from feeding period 1 to 2. Durunna et al. (Reference Durunna, Mujibi, Goonewardene, Okine, Basarab, Wang and Moore2011) proposed that such re-ranking was because of 1. BW and feed intake measurement error, 2. animal variation in response to compensatory gain, 3. animal variation in efficiency with animal maturity and 4. animal variation in diet digestibility because of differences in feeding behavior, rate of passage and rumen microbial population. Preliminary data from the Lacombe Research Centre, Canada, also confirms the moderate to strong repeatability of RFI over different stages of the animal's life (Basarab, 2012; unpublished). Replacement heifers identified as −RFIfat and +RFIfat when they were 8 to 12 months of age and fed a 90% : 10% barley silage and barley grain diet (as fed; −0.373 v. 0.365 kg DM/day) were also −RFIfat and +RFIfat when measured again as 4 to 7 year old cows and fed a 70% : 30% grass hay and barley straw cube diet (as fed; −0.375 v. 0.459 kg DM/day). Thus, these results indicate that RFI, and presumably RG and RFI–RG, are consistent across different stages of an animal's life.
RFI and related traits
Growth, carcass traits, FCR and feed intake
There are numerous studies examining the relationships of RFI with growth and carcass traits in cattle (Arthur et al., Reference Arthur, Renand and Krauss2001a and Reference Arthur, Archer, Johnson, Herd, Richardson and Parnell2001b; Richardson et al., Reference Richardson, Herd, Oddy, Thompson, Archer and Arthur2001; Herd et al., Reference Herd, Arthur, Hegarty and Archer2002; Basarab et al., Reference Basarab, Price, Aalhus, Okine, Snelling and Lyle2003; Nkrumah et al., Reference Nkrumah, Crews, Basarab, Price, Okine, Wang, Li and Moore2007; Crowley et al., 2010 and Reference Crowley, Evans, Mc Hugh, Pabiou, Kenny, McGee, Crews and Berry2011) including a recent review by Hill and Ahola (Reference Hill and Ahola2012). Briefly, RFI is not related to pre- and post-weaning growth, body size and slaughter weight in beef cattle and the phenotypic and genetic correlations are near zero. Carcass traits are also poorly correlated to RFI (Hill and Ahola, Reference Hill and Ahola2012), though some studies have reported a low-to-moderate correlation between RFI and carcass fatness (r p = 0.25 for grade fat; r p = −0.22 for lean meat yield; Nkrumah et al., Reference Nkrumah, Basarab, Price, Okine, Ammoura, Guercio, Hansen, Li, Benkel, Murdoch and Moore2004) and RFI and marbling (r g = 0.17; Robinson et al., Reference Robinson, Oddy, Dicker and McPhee2001). However, when RFI is adjusted for body fatness using ultrasound backfat thickness (RFIfat), the correlations were near zero (Basarab et al., 2003 and 2007). Thus, selection for low RFIfat breeding stock will have little to no effect on progeny growth, frame size, mature size or carcass characteristics.
RFI is moderately to highly correlated with feed intake (r p = 0.47 to 0.72; r g = 0.69 to 0.79; Arthur et al., Reference Arthur, Renand and Krauss2001a and Reference Arthur, Archer, Johnson, Herd, Richardson and Parnell2001b; Herd et al., Reference Herd, Arthur, Hegarty and Archer2002; Basarab et al., Reference Basarab, Price, Aalhus, Okine, Snelling and Lyle2003; Nkrumah et al., Reference Nkrumah, Crews, Basarab, Price, Okine, Wang, Li and Moore2007; Kelly et al., Reference Kelly, McGee, Crews, Sweeney, Boland and Kenny2010b) and FCR (r p = 0.46 to 0.70; r g = 0.66 to 0.88; Arthur et al., Reference Arthur, Renand and Krauss2001a and Reference Arthur, Archer, Johnson, Herd, Richardson and Parnell2001b; Basarab et al., Reference Basarab, Price, Aalhus, Okine, Snelling and Lyle2003; Kelly et al., Reference Kelly, McGee, Crews, Sweeney, Boland and Kenny2010b). This implies that selection for −RFIfat will decrease feed intake at equal levels of BW, growth and body fatness, and will improve feed-to-gain ratio in feeder cattle and growing replacement heifers. These results also imply that selection for −RFIfat will decrease CH4 emissions (g/animal per day) since CH4 emissions are proportional to feed intake (Blaxter and Clapperton, Reference Blaxter and Clapperton1965; Beauchemin and McGinn, Reference Beauchemin and McGinn2006; IPCC, 2006). A comprehensive analysis of Australian and Canadian data spanning a wider range in enteric CH4 emissions, diet quality, animal type (lactating dairy cows; growing beef steers) and CH4 measurement technique (SF6 in whole animal chambers; whole animal chambers) revealed strong positive, linear relationships between CH4 emissions (g/day) and DMI (Australian, R 2 = 0.45, P < 0.001; Canadian, R 2 = 0.68, P < 0.001; Grainger et al., Reference Grainger, Clarke, McGinn, Auldist, Beauchemin, Hannah, Waghorn, Clark and Eckard2007; Figure 3). Generally, the higher the DMI the higher the daily enteric CH4 emissions as more substrate is available for rumen fermentation and more hydrogen is available for methanogenesis (Pinares-Patinõ et al., Reference Pinares-Patinõ, Baumont and Martin2003; Lovett et al., Reference Lovett, Stack, Lovell, Callan, Flynn, Hawkins and O'Mara2005; Beauchemin and McGinn, Reference Beauchemin and McGinn2006; Grainger et al., Reference Grainger, Clarke, McGinn, Auldist, Beauchemin, Hannah, Waghorn, Clark and Eckard2007).
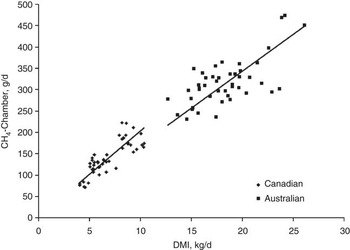
Figure 3 Relationship between CH4 emission determined in chambers and dry matter intake (DMI) for Australian and Canadian data. Lines are through the origin and have slope estimates of 17.06 for the Australian data (r 2 = 0.454, P < 0.0001) and 20.79 for the Canadian data (r 2 = 0.677, P < 0.0001). Adapted from Grainger et al. (Reference Grainger, Clarke, McGinn, Auldist, Beauchemin, Hannah, Waghorn, Clark and Eckard2007).
Since CH4 production rates (g/day) are proportional to DMI, they are often standardized to g CH4/kg DMI or expressed as a percentage of gross energy intake (GEI). Unfortunately, this has resulted in contradictory conclusions as CH4 production expressed as g/kg DMI or as a percent of GEI are not related to DMI (r < 0.20, P > 0.20; Beauchemin and McGinn, Reference Beauchemin and McGinn2006). In addition, when feed intake was expressed relative to maintenance, thus removing some variation because of daily feed intake, it was moderately and negatively associated with CH4 expressed as percent of GEI (r = −0.30; P = 0.04). Thus, increasing the intake level to 2.5 times maintenance decreased CH4 conversion by 19%, presumably because of reduced retention time in the rumen and lower acetate : propionate ratio (Beauchemin and McGinn, Reference Beauchemin and McGinn2006). Lower acetate and greater propionate production directs hydrogen away from CH4 production. Despite the strong positive relationship between daily CH4 production and DMI, there are numerous within- and between-animal factors that are unrelated to DMI since 54% to 70% of the total variation in daily CH4 production is associated with the animal despite being fed the same diet (Pinares-Patinõ et al., Reference Pinares-Patinõ, Baumont and Martin2003).
Feeding behavior
The genetic components of feeding behavior are important in animal breeding since they have economic and animal welfare implications, contribute to animal-to-animal variation in energetic efficiency (Herd et al., Reference Herd, Oddy and Richardson2004; Herd and Arthur, Reference Herd and Arthur2009; Kelly et al., Reference Kelly, McGee, Crews, Fahey, Wylie and Kenny2010a), digestibility and enteric methane emissions (Johnson et al., Reference Johnson, Huyler, Westberg, Lamb and Zimmerman1994; Harper et al., Reference Harper, Denmead, Freney and Byers1999). Feeding behaviors such as feeding duration, frequency and rate are moderately repeatable (r = 0.37 to 0.62: Kelly et al., Reference Kelly, McGee, Crews, Sweeney, Boland and Kenny2010b) and heritable (h 2 = 0.28 to 0.38; Nkrumah et al., Reference Nkrumah, Crews, Basarab, Price, Okine, Wang, Li and Moore2007). Furthermore, research from Australia (Robinson and Oddy, Reference Robinson and Oddy2004), Canada (Basarab et al., Reference Basarab, McCartney, Okine and Baron2007; Nkrumah et al., Reference Nkrumah, Crews, Basarab, Price, Okine, Wang, Li and Moore2007; Montanholi et al., Reference Montanholi, Swanson, Palme, Schenkel, McBride, Lu and Miller2010; Durunna et al., Reference Durunna, Mujibi, Goonewardene, Okine, Basarab, Wang and Moore2011), Ireland (Kelly et al., Reference Kelly, McGee, Crews, Fahey, Wylie and Kenny2010a) and the United States (Golden et al., Reference Golden, Kerley and Kolath2008; Bingham et al., Reference Bingham, Friend, Lancaster and Carstens2009) have reported mostly moderate to strong positive correlations (r = 0.08 to 0.62) of RFI and RFIfat to feeding duration, frequency and eating rate. Several of these relationships are further illustrated in Figures 4 and 5 by the moderate correlation between RFIfat and feeding event frequency in replacement heifers fed a growing diet (r p = 0.16, P < 0.1) and cows fed straw : hay cubes (r p = 0.57, P < 0.001) tested for feed efficiency at the Lacombe Research Centre, Canada (Basarab, 2012, unpublished). Collectively, these results show that –RFIfat and +RFIfat cattle have distinctive diurnal patterns of feeding behavior, with inefficient cattle having 14% to 22% more daily feeding events than efficient cattle (Nkrumah et al., Reference Nkrumah, Crews, Basarab, Price, Okine, Wang, Li and Moore2007; Kelly et al., Reference Kelly, McGee, Crews, Fahey, Wylie and Kenny2010a), thus expending 2% to 5% more energy in feeding activities (Herd et al., Reference Herd, Oddy and Richardson2004; Basarab et al., Reference Basarab, Colazo, Ambrose, Novak, McCartney and Baron2011). There are also implications for the direct measurement of enteric CH4 as CH4 emissions from the rumen are closely associated with feeding patterns; higher after feeding than at ruminating or resting (Johnson et al., Reference Johnson, Huyler, Westberg, Lamb and Zimmerman1994). Thus, whole-day measurement repeated across many days (e.g. 35 days as recommended for DMI by Wang et al., Reference Wang, Nkrumah, Li, Basarab, Goonewardene, Okine, Crews and Moore2006) are required to accurately reflect animal-to-animal differences in enteric CH4 production.
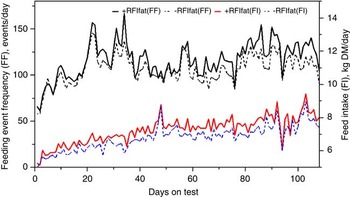
Figure 4 Daily average feeding event frequency (FF) and feed intake (FI) for residual feed intake (+RFIfat; n = 29) and −RFIfat (n = 32) beef heifers fed ad libitum a 78.2% barley silage and 21.8% barley grain diet (dry matter (DM) basis) over 108 days. Means (s.d.) are as follows: +RFIfat, FF = 116.3 events/day (33.2), black solid line; –RFIfat, FF = 106.3 events/day (33.2), black dashed line; +RFIfat, FI = 7.27 kg DM/day (1.34), red solid line; –RFIfat, FI = 6.81 kg DM/day (1.25), blue dashed line (Basarab, 2012, unpublished).
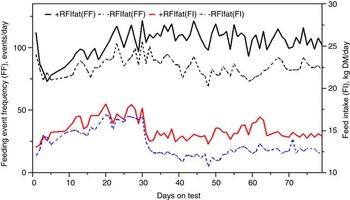
Figure 5 Daily average feeding event frequency (FF) and feed intake (FI) for residual feed intake (+RFIfat; n = 23) and –RFIfat (n = 17) beef cows fed a hay-straw cube over 79 days (25% straw: 75% grass hay-alfalfa mix, dry matter (DM) basis). Means (s.d.) are as follows: +RFIfat, FF = 105.3 events/day (21.0), black solid line; –RFIfat, FF = 85.5 events/day (17.9), black dashed line; +RFIfat, FI = 15.10 kg DM/day (1.55), red solid line; –RFIfat, FI = 13.32 kg DM/day (2.42), blue dashed line. Ad libitum and about 85% restricted feeding occurred from days 1 to 30 and 31 to 79, respectively (Basarab, 2012, unpublished).
In a recent review on feed efficiency and animal robustness, Rauw (Reference Rauw2012) hypothesized that because efficient animals have decreased feeding event duration and frequency, they are less able to adapt to changes in environment conditions. However, observation of beef cows reared under extensive Canadian winter conditions has shown that cows that produced –RFI calves actually had higher backfat thickness with no differences in lifetime productivity compared with their herd mates that produced +RFI calves (Basarab et al., Reference Basarab, McCartney, Okine and Baron2007). In addition, –RFIfat heifers calving for the first time had lower calf death within 30 days of birth than +RFIfat heifers, suggesting that calves born to efficient cows have improved early life survival possibly because of lower maintenance requirements and more available nutrients for accumulation of body fat, better uterine environment (Basarab et al., 2007 and 2011) and possibly improved calf passive immunity status. Indeed, recent unpublished research from the Lacombe Research Centre confirm these findings in that –RFIfat cows gained more body fat and BW than +RFIfat cows when both groups swath grazed forages for the first time during Canadian winters where night time ambient temperatures dropped below −20°C and animals grazed through the snow from November to March. Previous to this, both –RFI and +RFI young cows had been wintered together in smaller holding areas and fed barley silage to meet their nutritional requirements. Thus, while efficient cattle have lower feeding event duration and frequency and lower feed intake this does not mean that –RFIfat animals cannot compete or acquire forages during extensive grazing. Instead, it may imply that efficient animals are more adaptable and less susceptible to stress than +RFIfat or inefficient animals.
Fertility and cow productivity
The relationships of RFI or RFIfat on fertility and productivity in heifers and cows have recently been reviewed by Basarab et al. (Reference Basarab, Fitzsimmons, Whisnant and Wettemenn2012b). Briefly, −RFI and +RFI cows and heifers were similar in culling, pregnancy, calving and weaning rate, calving pattern, calf birth weight, level of dystocia and kilogram of calf weaned per mating opportunity (Arthur et al., Reference Arthur, Herd, Wilkins and Archer2005; Basarab et al., 2007 and 2011), however, −RFI cows calved 5 to 6 days later in the year than +RFI cows suggesting a delay in the onset of first estrus that may have delayed conception during the first breeding season (Arthur et al., Reference Arthur, Herd, Wilkins and Archer2005; Basarab et al., Reference Basarab, McCartney, Okine and Baron2007; Shaffer et al., Reference Shaffer, Turk, Wagner and Felton2011). When RFI was adjusted for body fatness (final off-test backfat thickness; RFIfat) no differences were observed in percentage of −RFIfat and +RFIfat heifers reaching puberty by 10, 11, 12, 13, 14 or 15 months of age nor in the percentage of calves born by day 28 of the calving season (86.5 v. 92.0, P = 0.28; Basarab et al., Reference Basarab, Colazo, Ambrose, Novak, McCartney and Baron2011 and Reference Basarab, Fitzsimmons, Whisnant and Wettemenn2012b). Calving difficulty, age at first calving, calf birth weight, calf pre-weaning ADG, calf actual and 200-day weaning weight and heifer productivity, expressed as kg calf weaned per heifer exposed to breeding, were also similar between −RFIfat and +RFIfat heifers. Fertility of young bulls, as measured by scrotal circumference, breeding soundness evaluation, calves born per sire and semen characteristics, for the most part has been unrelated to RFI, though several weak associations (r p = 0.13 to 0.21; P < 0.05) have been observed with sperm morphology and motility (Hafla et al., Reference Hafla, Lancaster, Carstens, Forrest, Fox, Forbes, Davis, Randel and Holloway2012; Wang et al., Reference Wang, Colazo, Basarab, Goonewardene, Ambrose, Marques, Plastow, Miller and Moore2012). These observations may also reflect the need to adjust RFI for off-test ultrasound backfat thickness and feeding behaviors in an effort to prevent the selection for later maturing bulls.
Basarab et al. (2007 and 2011) also reported a lower calf death loss in −RFIfat compared with +RFIfat heifers and cows, and suggested that the improved early life survival of progeny from −RFI mothers may be because of their improved feed efficiency resulting from more available nutrients and a better uterine environment compared with +RFI mothers. In addition, mothers that produced −RFI progeny consistently had 2 to 3 mm more backfat thickness, on average, over the 12th and 13th ribs and lost less weight during early lactation (pre-calving to pre-breeding) than mothers that produced +RFI progeny, thus indicating that the lower maintenance requirement of −RFI heifers and cows results in the accumulation of body fat or the loss of less body fat and weight during stressful environmental periods.
RFI, methane production and digestibility
Reduction of CH4 emissions from ruminants is important because it represents 2% to 12% loss of feed energy by cattle (Johnson et al., Reference Johnson, Huyler, Westberg, Lamb and Zimmerman1994; Johnson and Johnson, Reference Johnson and Johnson1995) and is linked to global warming. There are numerous opportunities for nutritional and microbial manipulation to reduce CH4 emissions in ruminants and these have been extensively reviewed by Beauchemin et al. (Reference Beauchemin, Kreuzer, O'Mara and McAllister2008) and McAllister and Newbold (Reference McAllister and Newbold2008). Enteric CH4 is produced during fermentation of feeds in both the reticulorumen and hindgut, with 95% to 98% excreted through the esophagus and lungs via brief 5 to 30 s eructations, burps and/or breaths and 2% to 5% via flatus (Johnson and Johnson, Reference Johnson and Johnson1995; Grainger et al., Reference Grainger, Clarke, McGinn, Auldist, Beauchemin, Hannah, Waghorn, Clark and Eckard2007). This leads to large fluctuations within and between days, and from animal-to-animal in CH4 production (Beauchemin and McGinn, 2005 and 2006; Jones et al., Reference Jones, Phillips, Naylor and Mercer2011; Pinares-Patinõ et al., Reference Pinares-Patinõ, McEwan, Dodds, Cárdenas, Hegarty, Koolaard and Clark2011). Methane production has low-to-moderate heritability (h 2 = 0.13 to 0.38; Crowley et al., Reference Crowley, McGee, Kenny, Crews, Evans and Berry2010; de Haas et al., Reference De Haas, Windig, Calus, Dijkstra, de Haan, Bannink and Veerkamp2011; Pinares-Patinõ et al., Reference Pinares-Patinõ, McEwan, Dodds, Cárdenas, Hegarty, Koolaard and Clark2011), low-to-moderate repeatability (r = 0.16 to 0.55; Robinson et al., Reference Robinson, Goopy, Hegarty and Vercoe2010; Pinares-Patinõ et al., Reference Pinares-Patinõ, McEwan, Dodds, Cárdenas, Hegarty, Koolaard and Clark2011) and is positively correlated to RFI (r p = 0.35 to 0.44; Nkrumah et al., Reference Nkrumah, Okine, Mathison, Schnid, Li, Basarab, Price, Wang and Moore2006; Hegarty et al., Reference Hegarty, Goopy, Herd and McCorkell2007), suggesting that it may be possible to breed for lower CH4 production and/or yield (g CH4/day; g CH4/kg DMI) either by directly selecting for a lower CH4 phenotype or indirectly by selecting for low RFIfat. The latter approach will be the emphasis of the following discussion.
A review of the scientific literature revealed three basic hypotheses as to why −RFI or −RFIfat animals have lower CH4 production and/or yield (Ørskov et al., Reference Ørskov, Fraser, Mason and Mann1988; Nkrumah et al., Reference Nkrumah, Okine, Mathison, Schnid, Li, Basarab, Price, Wang and Moore2006; Hegarty et al., Reference Hegarty, Goopy, Herd and McCorkell2007; Pinares-Patinõ et al., Reference Pinares-Patinõ, McEwan, Dodds, Cárdenas, Hegarty, Koolaard and Clark2011; Waghorn and Hegarty, Reference Waghorn and Hegarty2011; Rius et al., Reference Rius, Kittelmann, Macdonald, Waghorn, Janssen and Sikkema2012; Gomes et al., Reference Gomes, Sainz and Leme2013). The first hypothesis is primarily feed intake driven. Efficient animals have lower feed energy intake at equal levels of production, BW and fatness and lower CH4 and manure production since CH4 production is either 6.5% or 4% of GEI (<85% v. ≥85% concentrates in the diet) and manure production and N excretion is predicted based on DMI, and total digestible nutrient, CP and ash content of the diet (Herd et al., Reference Herd, Arthur, Hegarty and Archer2002; IPCC, 2006). This hypothesis assumed no effects of RFI phenotypes on digestibility or CH4 yield (g/kg DMI; % of GEI). The second hypothesis is also feed intake driven but assumed that feed intake affects retention time of digesta in the rumen and rumen volume such that longer retention times and higher rumen volumes are associated with increased CH4 production, likely because of increased digestion of structural carbohydrates and a greater supply of hydrogen for methanogens (Nkrumah et al., Reference Nkrumah, Okine, Mathison, Schnid, Li, Basarab, Price, Wang and Moore2006; Hegarty et al., Reference Hegarty, Goopy, Herd and McCorkell2007; Gomes et al., Reference Gomes, Sainz and Leme2013). Any small differences in DM or N digestibility (1 to 2 percentile points) are attributed to decreased metabolizability of the diet and increased heat increment of feeding at higher levels of feed intake above maintenance (Ferrell and Jenkins, Reference Ferrell and Jenkins1985). The third hypothesis suggested that inherent differences in feeding behavior and activity as previously discussed, feed intake and ruminal retention time results in a host-mediated response in microbial communities (bacteria, archaea, ciliate protozoa and fungi) favoring improved DM and N digestibility (Russell and Gahr, Reference Russell and Gahr2000; Nkrumah et al., Reference Nkrumah, Okine, Mathison, Schnid, Li, Basarab, Price, Wang and Moore2006). Higher ruminal fermentation rates favor a shift in acetate to propionate, thus decreasing the hydrogen available for methanogens.
Only a few studies have actually measured whole or near-whole day CH4 production from cattle differing in RFI. Measurement techniques have included indirect calorimetry where CH4 was measured continuously over 16 to 24-h periods (Nkrumah et al., Reference Nkrumah, Okine, Mathison, Schnid, Li, Basarab, Price, Wang and Moore2006; Montanholi et al., Reference Montanholi, Swanson, Vander Voort, Smith, Haas and Miller2011), SF6 as a tracer gas where CH4 was measured in a series of 10-day measurement periods (Hegarty et al., Reference Hegarty, Goopy, Herd and McCorkell2007), respiration chambers over 48 hs (Waghorn and Hegarty, Reference Waghorn and Hegarty2011) and open path Fourier Transform Infrared spectrophotometry over two 6-day periods (summer and winter, Western Australia; Jones et al., Reference Jones, Phillips, Naylor and Mercer2011). Diets varied from high grain fed to steers to low- and high-quality forage fed to cows. In three of these studies, −RFI steers consumed less feed and produced 24.6% to 27.5% less CH4 than +RFI steers (Canadian, University of Alberta data, 97.5 v. 129.3 g CH4/day, P = 0.04; Canadian, University of Guelph data, 493.4 v. 680.2 ml CH4/min, P = 0.08; Australian data, 142 v. 190 g CH4/day, P = 0.01). When expressed as % of GEI or per kg DMI, only the Canadian data reported lower methane yields for –RFI as compared with +RFI steers (3.19% v. 4.28% of GEI, P = 0.04). The cow results were more variable and ranged from no difference between RFI phenotypes in CH4 production (Waghorn and Hegarty, Reference Waghorn and Hegarty2011) to 23.1% less CH4 produced for −RFI cows and their calves grazing high quality (81% digestibility) annual pasture during the winter (0.34 ± 0.017 v. 0.46 ± 0.023 g CH4/kg live weight (LW)/day, P < 0.05; 13.1 ± 1.63 v. 14.0 ± 1.50 kg DM/500 kg LW/day, P>0.1; Jones et al., Reference Jones, Phillips, Naylor and Mercer2011). In this same study, RFI groups were similar in CH4 production when the cows were pregnant and grazing poor quality (55% digestibility) annual pasture during the summer (0.26 ± 0.018 v. 0.26 ± 0.013 g CH4/kg cow LW/day, P > 0.1; 10.2 ± 0.27 v. 10.7 ± 0.26 kg DM/500 kg LW/day; P > 0.1). The lack of differences in CH4 emissions between the RFI phenotypes during the summer may be attributed to the low CP content (7.1%) of the poor quality forage as 8% to 9% CP is required to meet the nitrogen requirements for the rumen microbes (NRC, 2000), thus affecting DMI and digestibility. The lack of difference in DMI between –RFI and +RFI cows may also be because of the large error associated with the mass balance method used to assess forage intake.
Similarly, only a few studies have measured DM and/or N digestibility in cattle differing in RFI (Nkrumah et al., Reference Nkrumah, Okine, Mathison, Schnid, Li, Basarab, Price, Wang and Moore2006; Cruz et al., Reference Cruz, Rodriguez-Sanchez, Oltjen and Sainz2010; Lawrence et al., Reference Lawrence, Kenny, Earley, Crews and McGee2011; Rius et al., 2012). In the study by Nkrumah et al. (Reference Nkrumah, Okine, Mathison, Schnid, Li, Basarab, Price, Wang and Moore2006), DM and CP digestibility tended to be greater for –RFI compared with +RFI steers, with medium RFI steers being intermediate (75.3% v. 73.4% v. 70.9% for DM digestibility, P = 0.10; 74.7% v. 73.5% v. 69.8% for CP digestibility, P = 0.09). In addition, RFI was positively associated with DMI, feeding event duration and bunk attendance, and feeding event duration was negatively related to DM (r = −0.55) and CP (r = −0.47) digestibility, indicating that lower feeding durations were associated with lower DMI and improved digestibility. These results are in agreement with Rius et al. (Reference Rius, Kittelmann, Macdonald, Waghorn, Janssen and Sikkema2012) where 16 ruminally cannulated (eight –RFI and eight +RFI) early lactating Holstein-Friesian heifers were fed ad libitum fresh vegetative ryegrass during an 8-day digestibility trial where total intake of nutrients and outputs of milk, feces and urine were determined. Efficient (−RFI) cows had greater apparent N digestibility (77.2% v. 75.5%, P = 0.02) and tended to have greater DM (78.5% v. 77.3%, P = 0.07) and organic matter (81.1% v. 80.1%, P = 0.08) digestibility than +RFI cows. Gomes et al. (Reference Gomes, Sainz and Leme2013), after a 5-day adaptation period, sampled feed and orts and collected total feces and urine throughout a 48-h period on 12 –RFI and 12 +RFI steers fed a finishing diet. Non-significant numeric differences between RFI phenotypes were observed in apparent DM digestibility (−RFI, 75.2%; +RFI, 72.3%; P = 0.18), nitrogen retention (−RFI, 60.0 g/day; +RFI, 53.2 g/day; P = 0.34) and nitrogen excretion (−RFI, 97.3 g/day; +RFI, 108.8 g/day; P = 0.31). Cruz et al. (Reference Cruz, Rodriguez-Sanchez, Oltjen and Sainz2010) and Lawrence et al. (Reference Lawrence, Kenny, Earley, Crews and McGee2011) used internal markers (lignin and acid-insoluble ash) and fecal grab samples to estimate digestibility reported no differences in N or DM digestibility between –RFI and +RFI cattle.
Thus, there is direct evidence indicating that –RFI cattle will produce less CH4 (g CH4/day) than +RFI cattle, primarily through lower feed intake at equal levels of production, BW and fatness. However, the evidence for greater feed efficiency in –RFIfat cattle due, at least partially to an enhanced capacity to digest feeds is controversial as CH4 yield (g CH4/kg DMI or as % of GEI) between RFI phenotypes were inconsistent among studies. The relationship between RFI and DM and N digestibility is also unclear, though it is known that higher DMI will increase CH4 production, but shorter rumen retention time associated with higher DMI will lower digestibility by 1 to 4 percentage points depending on the level of feed intake above maintenance, lower CH4 yield (g/kg DMI) and increase heat increment of feeding (Ferrell and Jenkins, Reference Ferrell and Jenkins1985; NRC, 2000; Waghorn and Hegarty, Reference Waghorn and Hegarty2011). Thus, the small differences in digestibility between –RFI and +RFI cattle observed in the above mentioned studies are more likely because of differences in feed intake rather than because of any real differences in ability to digest feed.
RFI, rumen microbiota and methane emissions
This section examines the third hypothesis which implies that inherent differences between RFI phenotypes in feed intake and feeding behaviors results in host-mediated alterations in microbial profiles and fermentation patterns. Indeed, the rumen bacterial profiles and not the total numbers of bacterial cells of −RFI steers are different than those in +RFI cattle when fed growing (Hernandez-Sanabria et al., Reference Hernandez-Sanabria, Guan, Goonewardene, Li, Fujibi, Stothard, Moore and Leon-Quintero2010) and finishing diets (Guan et al., Reference Guan, Nkrumah, Basarab and Moore2008; Hernandez-Sanabria et al., Reference Hernandez-Sanabria, Guan, Goonewardene, Li, Fujibi, Stothard, Moore and Leon-Quintero2010). A recent study by Irish researchers further confirmed segregation of rumen microbial profiles in forage-fed beef heifers with different RFI values (Carberry et al., Reference Carberry, Kenny, Han, McCabe and Waters2012). In this study, abundance of some Prevotella species was higher (P < 0.0001) in +RFI animals. Further, some Prevotella sp. have been associated with increased butyrate production, poorer FCR and a lower straight-chain to branched-chain volatile fatty acids (VFA) ratio (Hernandez-Sanabria et al., Reference Hernandez-Sanabria, Guan, Goonewardene, Li, Fujibi, Stothard, Moore and Leon-Quintero2010). Conversely, other Prevotella species were higher in −RFI animals (Ghoshal et al., Reference Ghoshal, Hernandez-Sanabria, Zhou, Stothard and Guan2012). The Prevotella genus predominates (up to 60% of total population) in the rumen under many dietary conditions (Stevenson and Weimer, Reference Stevenson and Weimer2007) and plays a role in degradation and utilization of starch (Cotta, Reference Cotta1992) and proteins (Wallace et al., Reference Wallace, McKain and Broderick1993). Therefore, it is not surprising that some species belonging to this genus are associated with better fermentation profiles and feed efficiency. In addition, some bacterial species such as Clostridium sp. are associated with lower straight-chain to branched-chain VFAs and improved FCR (Hernandez-Sanabria et al., Reference Hernandez-Sanabria, Guan, Goonewardene, Li, Fujibi, Stothard, Moore and Leon-Quintero2010). Branched-chain VFAs are derived from branched-chain amino acids (e.g. leucine, valine and isoleucine) and the ratio of straight-chain to branched-chain VFA is an indicator of amino acid fermentation (Hernandez-Sanabria et al., Reference Hernandez-Sanabria, Guan, Goonewardene, Li, Fujibi, Stothard, Moore and Leon-Quintero2010). Thus, Clostridium sp. can ferment amino acid and peptide to produce ammonia (Eschenlauer et al., Reference Eschenlauer, McKain, Walker, McEwan, Newbold and Wallace2002), suggesting that the increased amino acid metabolism plays an important role in feed efficiency. Furthermore, some novel ruminal species have been observed to be associated with specific fermentation functions. Pelotomaculum thermopropionicum, for example, is an anaerobic propionate-oxidizing bacterium (Imachi et al., Reference Imachi, Sekiguchi, Kamagata, Hanada, Ohashi and Harada2002) that has been associated with low propionate and improved FCR (Hernandez-Sanabria et al., Reference Hernandez-Sanabria, Guan, Goonewardene, Li, Fujibi, Stothard, Moore and Leon-Quintero2010). Propionate, one of the major VFA in the rumen, is the major gluconeogenic precursor whose concentration is dependent on digestible energy intake (Stewart et al., Reference Stewart, Flint and Bryant1997).
Recent high throughput sequencing studies have revealed that the rumen has greater than 700 bacterial species (Brulc et al., Reference Brulc, Antonopoulos, Miller, Wilson, Yannarell, Dinsdale, Edwards, Frank, Emerson, Wacklin, Coutinho, Henrissat, Nelson and White2009), suggesting that there is limited value in studying the rumen microbial community at the taxonomy level and emphasizing the need to examine enzymes involved in carbohydrate and amino acid metabolism. Use of a metagenomic approach has revealed variation in ruminal microbial enzymes between –RFI and +RFI animals (Ghoshal et al., Reference Ghoshal, Hernandez-Sanabria, Zhou, Stothard and Guan2012). The activity of enzymes involved in benzoate metabolism was higher in the rumen of +RFI steers while enzymes involved in carbazol degradation were higher in −RFI steers. The 4-carboxymuconolactone decarboxylase enzyme plays a role in benzoate degradation which leads to overproduction of CO2 ultimately leading to increased production of CH4 in +RFI steers. Further, carbazol degradation may lead to a decreased production of CO2 which lowers the substrate necessary for CH4 emission.
The methanogenic process plays a role in regulating the overall fermentation in the rumen by removing H2 that benefits the donors by providing an electron sink for reducing equivalents to minimize the partial pressure of H2 in the rumen (Wolin et al., Reference Wolin, Miller and Stewart1997; Russell, Reference Russell2002). Methanogens are found in a symbiotic relationship with ruminal bacteria (Wolin et al., Reference Wolin, Miller and Stewart1997) and protozoa (Lange et al., Reference Lange, Westermann and Ahring2005). Only a few species of methanogens have been successfully isolated from the rumen and identified because of their stringent requirements for growth. Recent studies using molecular-based approaches have reported that the differences in methanogenic profiles, not the total population of methanogens may be associated with feed efficiency of cattle (Zhou et al., 2009 and 2010). The methanogenic community in +RFI cattle has been observed to be more diverse than that in –RFI cattle, and the differences at genus, species, strain and genotype levels were associated with feed efficiency when fed low energy diets. Several researchers have examined the relationship between methanogenic populations and variation in feed efficiency. The observed proportion of Methanosphaera stadtmanae and Methanobrevibacter sp. AbM4 in the rumen were higher in +RFI compared with −RFI cattle (Zhou et al., Reference Zhou, Hernandez-Sanabria and Guan2009). M. stadtmanae utilizes methanol for CH4 synthesis (Miller and Wollin, 1985), and M. sp. AbM4 is closely related to Methanobrevibacter smithii, a species that utilizes acetate for CH4 production (Zhou et al., Reference Zhou, Hernandez-Sanabria and Guan2009). These results suggest increases in populations that shift more organic substrates to CH4 biosynthesis pathways, which may contribute to low feed efficiency. In addition, the association between methanogen profiles and RFI was affected when the animals were switched from low to high-energy diets (Zhou et al., Reference Zhou, Hernandez-Sanabria and Guan2010). These results suggest that the diversity of the methanogenic community affects CH4 emissions and feed efficiency in cattle. However, CH4 was not measured in the above studies, and trials to link the methanogenic profiles with the CH4 emissions are necessary to verify and elucidate the roles of methanogen in the animals with different feed efficiency. Furthermore, the interaction between methanogens and bacteria, as well as protozoa is largely unknown.
Breeding for low RFI and reduced CH4 emissions
Actual selection for RFI has been conducted at the Trangie Agricultural Research Centre, NSW, Australia, with a direct yearly response because of selection of −0.125 kg DM/day compared with no selection for RFI (Arthur et al., Reference Arthur, Archer, Herd and Melville2001c). However, given that multi-trait breeding goals will be pursued by the industry, Alford et al. (Reference Alford, Hegarty, Parnell, Cacho, Herd and Griffith2006) assumed an annual rate of response in RFI of −0.08 kg DM/day (0.8%/year) in a 25-year simulation assessing the impact on enteric CH4 abatement for the Australian beef industry. Cumulative CH4 reduction over 25 years was 568 100 t which was equivalent to an annual reduction of 568 100 t CO2e/year or $8.5 million/year in carbon credits assuming a global warming potential of 25 for CH4 and a value of $15/t for CO2e. This study did not account for reduced manure CH4 and N20, cropping N2O and energy CO2 because of reduced feed requirements resulting from lower RFI. The HOLOS whole-farm model (Little et al., Reference Little, Linderman, Maclean and Janzen2008) which does account for enteric CH4, manure CH4 and N20, cropping N2O and energy CO2 was used to predict the reduction in total GHG emissions that would occur after 25 years of selection for −RFI compared with a baseline 120-cow herd ( four breeding bulls) not selected for RFI. Animal and cropping data from Beauchemin et al. (Reference Beauchemin, Janzen, Little, McAllister and McGinn2010), percentage RFI reduction (% of base year) for different age cohorts in a commercial herd over 25 years from Alford et al. (Reference Alford, Hegarty, Parnell, Cacho, Herd and Griffith2006), and updated global warming potentials of 25 for CH4 and 298 for N2O were used for this HOLOS simulation. After 25 years of selection for −RFI, the efficient 120-cow beef herd had lower GHG emissions by 101 t CO2e per year or 0.844 t CO2e per cow per year compared with the average 120-cow herd. In addition, the feed nitrogen use efficiency and carbon footprint of the efficient herd were improved by 17% to 22% and 14%, respectively, compared with the cow herd not selected for RFI (19.82 v. 23.06 kg CO2e/kg carcass beef) and the total farm area decreased by 13% because we use less farm grown resources to produce an equivalent amount of animal product. These estimates of GHG reduction are conservative as improved DM digestibility in –RFI cattle and improved accuracy and rate of genetic change resulting from genomic enhanced breeding values were not considered.
There is on-going debate as to the best methods to use when selecting for improved RFI. The merit of including RFI in the breeding goal over and above just feed intake exists to a different extent depending on the breeding tools available to a particular group of producers. For example, where a multiple trait selection index is used, including RFI as a goal trait may hold little or no advantage to using the components (feed intake, ADG, metabolic LW and back fat) as goal traits along with proper weightings. However, RFI may be a ‘cleaner’ trait than feed intake to include as it is essentially feed intake corrected for different energy uses at the time of measurement. Similarly, since RFI is effectively a selection index, a feed efficiency sub-index can be derived using restricted selection index methodology (Eisen, Reference Eisen1977). Ultimately, including either feed intake or RFI in a breeding goal is mathematically equivalent given that all parameters are known without error (Van der Werf, Reference Van der Werf2004).
In a situation where only expected progeny difference (EPD) are generated for different traits, and selection pressure for each trait is determined by the producer, selecting for multiple traits simultaneously as well as attempting to select for feed efficiency without a direct measure may be a lot to ask. Selecting for reduced feed intake alone may be equivalent as producers would inherently select for increased weaning weight but the notion of selecting for reduced feed intake (if selection pressure was left to the producer) may seem improper especially when there is a ‘feed for fertility’ mantra in existence. In this instance, deriving EPD for RFI is useful. In a third situation, where no genetic evaluation is available to the producer, RFI can be used to rank animals for feed efficiency within population. It should also be taken into consideration when proposing RFI, RIG or residual intake and gain to producers as a selection criterion that it is a difficult concept to grasp (Wulfhorst et al., Reference Wulfhorst, Ahola, Kane, Keenan and Hill2010) and this may lower industry acceptance of residual feed efficiency measures. It is positive to note that RFI is favorably genetically correlated or not correlated with the majority of other production and carcass quality traits as previously discussed and selection for RFI will reduce GHG emissions in ruminants.
Conclusion
Selection for feed efficiency through residual or its component traits (DMI, BW, ADG and backfat) in a multi-trait selection index will result in slow incremental improvement to feed efficiency and methane intensity, with few antagonistic effects on traits of economic importance.
Acknowledgements
This paper was published as part of a supplement to animal, publication of which was supported by the Greenhouse Gases & Animal Agriculture Conference 2013. The papers included in this supplement were invited by the Guest Editors and have undergone the standard journal formal review process. They may be cited. The Guest Editors appointed to this supplement are R. J. Dewhurst, D. R. Chadwick, E. Charmley, N. M. Holden, D. A. Kenny, G. Lanigan, D. Moran, C. J. Newbold, P. O'Kiely, and T. Yan. The Guest Editors declare no conflict of interest.