Appendix A Radio Astronomy
Radio Waves
This is a book in which radio telescopes play a central role. While we think it is likely that most readers of our book are familiar with that subject, we would also guess that a minority of our audience (and most of the general population) do not know all that much about radio telescopes and the scientific revelations they have produced. Our aim here is to remedy this situation for our non-astronomer readers as briefly and non-technically as possible.
All astronomical telescopes share the same basic functional structure. Radio telescopes image celestial phenomena just as optical telescopes, but they do so in radio waves rather than visible light. Humans are simply not equipped to see radio waves. You can look through even a small telescope and see the mountains and craters of the Moon, but without complicated equipment, it is not so easy to detect even the strongest radio waves that reach Earth from interstellar space. Nonetheless, the underlying commonality of all telescopes is the result of the fact that the light by which we see turns out to be part of a fundamental and far grander phenomenon that is largely invisible to the eye, namely electromagnetic waves.
Visible light is an electromagnetic wave. The different colors of the light seen in a rainbow, are part of the same phenomenon, simply made up of light of slightly different wavelengths. More important, theory does not forbid electromagnetic waves of radically different wavelengths, ranging from the ultrashort (what we now call x-rays and gamma rays) to the comparatively long (what we now call the radio regime). Figure A.1 illustrates the bands of the electromagnetic spectrum.

Figure A.1 The electromagnetic spectrum from gamma rays to radio waves. Visible light occupies only a narrow slice compared with the radio band.
The characteristics of any wave are its wavelength (distance between successive wave peaks), its frequency (the number of waves passing a fixed point per second), and its speed. By definition, wavelength times frequency is equal to speed. The speed (in a vacuum) of electromagnetic waves, produced by accelerated charges, is the speed of light. The modern term “radio” usually includes all electromagnetic waves longer than the infrared. This is actually a huge span of wavelengths, running from about 100 km (approximately 60 miles) to around 1,000 millionths of a meter (1,000 microns, or about 1/25th of an inch). Obviously, dealing with such a large range of numbers can become very cumbersome, and for convenience, it is usual for scientists and engineers to subdivide the radio regime into smaller domains. Electromagnetic signals with wavelengths shorter than about 10 cm are commonly called microwaves, waves shorter than 1 cm are called millimeter waves, and waves shorter than 1 mm submillimeter waves.
Radio telescopes observe four types of cosmic radiation: synchrotron, bremsstrahlung, thermal, and spectral line. Figure A.2 illustrates the first of these mechanisms. Synchrotron radiation is produced by free electrons following spiral paths around magnetic field lines. The electrons are constantly accelerated while following these paths and they emit electromagnetic radiation which is strongest at low frequencies in the radio band. Radio astronomy began with the observation of synchrotron radiation. It was the discovery of such radiation from the center of our Galaxy by Karl Jansky in 1933 that marks the birth of radio astronomy. Figure A.3 is an image of synchrotron emission captured by the Very Large Array (VLA) of the radio galaxy Hercules A.

Figure A.2 Synchrotron radiation produced by an electron spiraling in a magnetic field.
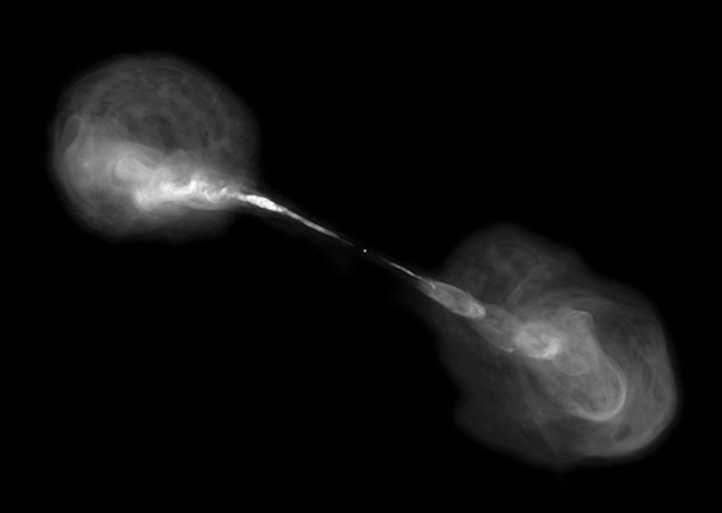
Figure A.3 The radio galaxy Hercules A as imaged by the VLA. The narrow jets emanate from a black hole in the center of the galaxy and end in the large lobes. The synchrotron emission of the radio image reveals a structure not seen in visible light; the optical emission from the galaxy itself would only show a small dot in the center.
Bremsstrahlung radiation is emitted from a plasma of ionized (largely) hydrogen atoms. The electrons are deflected in collisions with the protons, emitting radio waves. The process is illustrated in Figure A.4. The plasma is created in the interstellar medium by nearby hot, luminous stars. The Orion Nebula is a prominent example in our Milky Way Galaxy, shown in Figure A.5.

Figure A.4 An electron deflected by an ionized atom radiates bremsstrahlung radiation.

Figure A.5 The Orion Nebula imaged in bremsstrahlung radiation by the VLA. Such radiation implies a plasma of ionized atoms in this star-forming region.
Thermal radiation is produced by the random motions of electrons in a warm object; that is, any object with a temperature above absolute zero. All the room temperature objects in our daily environment, including our bodies, radiate in the infrared. At the higher temperatures found, for example, in flames, the radiation is visible; we see the glow of heated objects. Stars have temperatures that cause them to radiate in the visible light band and in the adjoining infrared and ultraviolet bands. The light thus recorded by an optical telescope is almost always starlight, and objects in the Solar System are seen in reflected light from the Sun. Images of our Galaxy and external galaxies are composed of a myriad of stars, as Galileo found when he turned his telescope to the Milky Way. In contrast, the cosmic background radiation, cool in temperature, is strongest at millimeter wavelengths. It is thermal radiation from the Big Bang. Thermal radiation from the dust in protoplanetary disks is seen in the images made by ALMA (see Chapter 10, Figures 10.1 and 10.2). ALMA, the subject of this book, is an instrument exquisitely designed for observations of thermal radiation to study the “cold universe.”
Spectral line emission occurs because the energy of atoms and molecules is confined to particular states or energy levels. For example, the tumbling motion of the carbon monoxide (CO) molecule cannot proceed at an arbitrary rate but must occur in quantum states labeled by a number that indicates their spin rate. Figure A.6 illustrates the source of three lowest frequency spectral lines of CO, namely transitions from the J = 1, 2, and 3 energy states to the next lowest energy state. Figure A.7 is an image1 of the galaxy M51 in the radiation from the J = 1 to J = 0 quantum state of CO.

Figure A.6 The three lowest frequency transitions between the quantized energy levels of CO: J = 1–0 at 115 GHz, J = 2–1 at 230 GHz, and J = 3–2 at 345 GHz. These frequencies of CO are the workhorse observational frequencies for ALMA, among the thousands of frequencies from the two hundred plus interstellar molecules.

Figure A.7 M51, the Whirlpool Galaxy, imaged in the radiation from the lowest frequency spectral line of carbon monoxide, CO, at 115 GHz. The image combines data obtained by CARMA and the Nobeyama 45 m Telescope.
Telescopes
In 1609, Galileo Galilei, who held the Chair of Mathematics at the University of Padua and who was helping to move the science of physics out of the swamp of Greek philosophy and into the realm of verifiable natural law, fabricated a small telescope. Galileo did not invent the telescope.2 However, he was, as far as is known, the first human being to turn a telescope on the night sky, explore what he found in a systematic way, and analyze and publish his findings. Galileo’s first telescope only magnified by a factor of three, but just a few months later he had built a 20-power instrument. Observations with it revealed the Moon to be a world unto itself, with mountains, craters, and what seemed to be (but were not) seas and oceans, and led to the discovery of the four largest moons of Jupiter; other observations showed that the planet Venus exhibits phases like those of the Moon, and that what appeared to the naked eye to be diffuse nebular patches of light in the Milky Way could be at least partially resolved into stars using his telescope. Galileo continued to improve his designs, and by 1611 further observations showed the Sun was not the perfect orb presumed by philosophers; instead, it had a surface harboring small, dark spots that revealed a slow rotation about its axis.
For the next 320 years, every astronomical telescope built on Earth was essentially an elaboration of Galileo’s first instrument, designed with one fundamental goal: to extend the reach of human vision. Beginning in the early twentieth century, astronomers began using new technologies to build instruments aimed at portions of the electromagnetic spectrum that lay beyond the narrow range of wavelengths directly visible to the human eye, and by the latter part of the twentieth century, astronomers had added gamma-ray, x-ray, ultraviolet, infrared, and radio telescopes to their kit.
Nevertheless, most modern radio telescopes share a common basic architecture: a large collecting element to gather and focus faint light from the sky. This is followed by a “receiving system” which detects and amplifies the light, digitizing and recording an image of the observations. This latter process is directly comparable to the way the lens, electronic sensor, and memory card of modern digital cameras operate to produce a digital image.
The generic structure of a typical radio telescope is quite similar to that of optical reflecting telescopes. Notably, there is a signal collecting element – usually a reflecting surface – that is functionally identical to the main mirror and subsidiary light-handling elements of an optical telescope. Radio waves that are captured by the reflector are then focused to go through a sequence of electronic systems that detect, amplify, digitize and finally record the captured signals, just as in the case of modern optical telescopes and digital cameras. Radio astronomers still use the descriptive term “receiver” for the electronics responsible for the initial stage of signal detection. An important common feature of most optical and radio telescopes arises because both types of instruments must have the ability to continuously follow astronomical sources across the sky in order to compensate for their apparent motion caused by the Earth’s rotation. Figure A.8 shows the functional outline of a single-dish radio telescope.

Figure A.8 Conceptual diagram of a single-dish radio telescope.
While single-dish radio telescopes typically have a close one-to-one correspondence with their optical counterparts functionally, sometimes more specialized radio telescope designs are required to overcome technical or physical performance limitations. Invariably, such designs will involve tradeoffs. We discuss one of these next.
Radio Interferometers
A particularly acute performance limitation of single-dish radio telescopes is their relatively poor resolution. The term resolution here simply means the detail that can be seen in an image. It is measured as the angle subtended by two barely discernable objects as seen by the observer. The resolution of any telescope that is not limited by atmospheric distortion is proportional to the wavelength of the electromagnetic waves it is designed to detect divided by the size of its main reflector. Since radio waves are defined by having enormously larger wavelengths than visible light waves, this means that a radio telescope must be enormously larger than any given optical telescope if it is to provide an image of comparable detail. Consequently, a single-dish radio telescope operating at a wavelength of 1 mm would have to be nearly 3 km in diameter if one wanted to produce radio images with the same level of detail as the Hubble Space Telescope.
Building a steerable, rigid, radio telescope dish 3 km in diameter is a structurally hopeless task. An antenna that size would have to be supported at its center, which would therefore have to sit on a pedestal or other support structure around 1.5 km in height. This is about twice the height of the Burj Khalifa, the tallest building yet built by the human race. More to the point, the largest steerable radio telescope in the world has a main dish around 100 m in diameter, close to the limiting size possible.3
It is this frustrating situation that led radio astronomers to develop instruments called imaging interferometers, which can provide the resolution of much larger radio telescopes by using many smaller radio antennas to at least partially fill the “footprint” of the larger antenna they are unable to build. Superficially, an imaging interferometer looks like an array of similar radio telescopes that observe the same source at the same time. This appearance masks a significant increase in complexity. In an interferometer, the individual telescope outputs are connected to a highly specialized computer, called a correlator, which combines the data streams to form an image having a resolution comparable to what would be produced by an antenna whose diameter is equal to the largest separation of the various small antennas in the array.
A price is paid for the gain in resolution. An imaging interferometer is in some ways a poor astronomer’s giant telescope, that is, one missing most of its reflecting surface. It is a “sparsely filled” aperture. While the notional giant antenna would catch all the radio waves that fall on its reflecting surface, a sparsely filled aperture of the same size can only collect signals that happen to fall on the parts of the giant antenna’s footprint that are occupied by smaller antennas. Thus, an imaging interferometer cannot match the sensitivity of the ideal giant telescope. There is also an important technical issue associated with the images produced by sparse arrays. Due to missing reflector elements relative to the ideal fully filled surface, there is irretrievable missing information needed to fully reconstruct radio images. Put another way, the images produced by radio interferometers are estimates of what a giant full aperture telescope would yield, and they require substantial off-line computer processing to attain their full potential.
NRAO’s VLA, built during the 1970s and dedicated in 1980, was the first large imaging interferometer. (Large interferometers are called arrays.) The VLA is shown in Figure A.9. Although interferometers are much more complex than single-dish telescopes, they have become indispensable instruments to modern radio astronomy. ALMA is an imaging radio interferometer, the product of a long history in the development of radio telescopes.

Figure A.9 Ten of the 27 antennas that make up the Very Large Array in New Mexico.
Misconceptions
Radio telescopes have nothing whatsoever to do with radio and television sets or their broadcasts, although all involve radio waves. But this misconception is remarkably common. A resident of Green Bank, West Virginia, once complained to the Observatory that the radio astronomers were watching her through her television set, which would have been a stunning technical achievement were it even possible. Another common misconception is that radio astronomers only listen for messages from extra-terrestrial civilizations. John Kraus, a pioneer in radio astronomy, implied just that when he named the telescope he used to search for such signals the “Big Ear.” The Search for Extra-Terrestrial Intelligence (SETI) does use radio telescopes to search for artificial signals, a sign of non-terrestrial life. In 1960, the first such search was conducted at NRAO’s Green Bank site using the Tatel Telescope, an 85 ft diameter antenna. The search was called Project Ozma4 and was conducted by NRAO staff members, led by Frank Drake. The movie Contact, based on the novel of the same name by Carl Sagan, showed the star Jodie Foster listening with headphones connected to the VLA. Radio astronomers today do not use headphones, nor do they “listen.” The popularity of the movie reinforced this notion in the general public’s concept of radio astronomy. But SETI is only a small aspect of radio astronomical activity. The vast majority of radio astronomers are studying the natural emission in radio waves from celestial objects, not searching for artificial emission transmitted by a distant civilization.
Appearances can lead to misconceptions. Satellite television and radio systems may employ ground stations that include one or more large dish-type antennas for downloading content, but these are not radio telescopes. Likewise, space agencies such as NASA and sister organizations elsewhere in the world that track and operate deep space missions send commands to their spacecraft and download scientific data using large antennas that resemble radio telescopes. Their mission and purpose is not radio astronomy. Not every antenna is a radio telescope.
Finally, some radio telescopes look nothing like a dish. This is particularly true at long radio wavelengths. A classic example is the antenna, shown in Figure A.10, that Karl Jansky used in 1932 to make his discovery of radio waves from the center of the Milky Way. This was the first detection of radio waves from a non-terrestrial source, the beginning of radio astronomy.

Figure A.10 Karl Jansky standing in front of his discovery making radio telescope.
In summary, beginning with Jansky, radio astronomy has revealed an entirely new view of the Universe, one rich in discoveries.5 ALMA is the most recent example of the power of radio telescopes to advance our understanding of cosmic phenomena. We can look forward to the era of the New Generation Very Large Array (ngVLA) and the Square Kilometer Array Observatory (SKAO), which will accomplish at lower frequencies what ALMA has done at higher frequencies.
Notes
1 Koda et al. (Reference Koda2009).
2 However, the telescope was a fairly recent invention in Newton’s time, as documented by two patent applications to the Dutch government that were never granted. By 1609, details of the simple design had been widely disseminated throughout Europe. The development of lenses and the telescope has been described by the Galileo Project. http://galileo.rice.edu/sci/instruments/telescope.html.
3 The US Naval Research Laboratory attempted to build a 600 ft diameter antenna in the late 1950s at a site in Sugar Grove, Virginia, near the NRAO Green Bank site. The project was a fiasco and it was canceled in 1962 by Defense Secretary Robert McNamara. See Kellermann, Bouten, and Brandt (Reference Kellermann, Bouton and Brandt2020) for a description of the project.
4 For a history of SETI, see Drake and Sobel (1992).
5 For an account of radio astronomy discoveries, see Kellerman and Bouton (2023).
Appendix B Millimeter/Submillimeter Telescopes
US Millimeter Telescopes
Here we briefly introduce and summarize a number of the most impactful single-dish millimeter wavelength telescopes constructed in the late twentieth century. These built the legacy of millimeter wave astronomy that led to ALMA.
University of Texas Millimeter Wave Observatory
Observations began with the 4.9 m diameter telescope of the Electrical Engineering Research Laboratory of the University of Texas in June of 1963, at its off-campus site at the J.J. Pickle Research Center in the northern outskirts of Austin, Texas. After measuring the brightness at 35, 70, and 94 GHz of a number of planets, the antenna was moved to Mt. Locke, the site of McDonald Observatory in the Davis Mountains of West Texas, where it was housed in an astrodome, shown in Figure B.1. In 1971, the antenna was “discovered” by Pat Thaddeus and one of the authors (Vanden Bout), who were observing on the McDonald 107 Inch Telescope. The antenna was idle. By the fall of 1972, a partnership began using the Millimeter Wave Observatory (MWO) to exploit the potential of the recently discovered interstellar CO. Bell Labs contributed the receivers, the Goddard Institute of Space Studies (GISS) purchased the klystron oscillators required for the receiver system, and Harvard College Observatory built the spectrometer or “backend.” The observing time was shared equally among the four partners. The MWO had a large number of observers from the United States and abroad, and MWO data have been included in at least 23 doctoral dissertations. Research tended to be concentrated on mapping individual CO sources in the Galaxy. Perhaps the most significant discovery was of jets of gas known as bipolar outflows that are the by-product of young stars, most importantly, the source known as L1551.1 The MWO was decommissioned in 1988. The reflecting surface of the 4.9 Meter Telescope is at the campus of the Instituto Nacional de Astrofísica, Óptica y Electrónica in Tonanzintla, near Puebla, Mexico2; the astrodome and mount have been moved to Sierra Negra, the site of the Large Millimeter Telescope (LMT).

Figure B.1 The MWO 4.9 Meter Telescope in its renovated astrodome equipped with an error-correcting subreflector.
Columbia University Mini Telescope
In 1974, Thaddeus and his group at GISS installed a 1.2 m diameter antenna in a dome on the roof of the Pupin Physics Building of Columbia University. The relatively small size of the antenna was deliberately chosen, as a smaller telescope with its wide field of view can rapidly construct maps of the CO distribution in the plane of the Milky Way. The “Mini” was highly successful, producing maps of Galactic CO, which led to numerous studies3 interpreting these maps and features within. Figure B.2 shows the Southern Mini, installed in 1982 at the Cerro Tololo Inter-American Observatory (CTIO) in Chile, which supplied the complementary data from the southern hemisphere. Observations of Galactic CO with the Southern Mini were conducted by Leo Bronfman of the U. Chile. The first Mini was moved to Harvard College Observatory in 1986 and its operation continues to the present under the leadership of Tom Dame.

Figure B.2 The Southern Mini in its astrodome at CTIO.
Aerospace Corporation Millimeter Telescope
The Aerospace Corporation is a research organization originally supported by the Air Force to conduct defense research. It also had a vigorous program of research in optical and x-ray astronomy through the 1970s when a 4.6 m diameter antenna for millimeter wavelength observing was constructed. The antenna resided on the roof of the Aerospace building just south of the Los Angeles International Airport. It had been built by the Rohr Corporation, shortly before Rohr built the NRAO 36 Foot Telescope. The proximity to the Pacific Ocean made for a less than desirable site for a millimeter telescope but it was adequate for observations at 3 mm wavelength. It made observations of planets, quasars, and the association of CO emission with individual objects in the Galaxy. A report of the activities in 1975 is typical of the observing programs4 which involved mapping CO emission from Galactic molecular clouds. A significant contribution of the Aerospace telescope was a map of the emission from the two isotopologues, 13CO and 12CO, in L134 which showed that the abundance of 13CO with respect to 12CO increased toward the center of the molecular cloud, as was predicted by models of isotopic fractionation in dense clouds. Anneila Sargent commuted from Caltech in Pasadena to make the observations for her PhD dissertation using the Aerospace antenna. The staff of the Aerospace Telescope included William Wilson, Eugene Epstein, and one of the authors (Dickman), who was in charge of operations.
Five College Radio Astronomy Observatory
The Five College Radio Astronomy Observatory (FCRAO) was organized in 1970 as a partnership between Hampshire, Amherst, Smith, Mount Holyoke Colleges, and the University of Massachusetts, Amherst. The first research project was the study of pulsars using a cluster of four “mini-Arecibo” telescopes, consisting of reflecting mesh draped between utility poles. The telescope was located in a wooded reserve at Quabbin Reservoir. This work led in time to the discovery by Hulse and Taylor, at the Arecibo Observatory, of a binary pulsar with a millisecond period. The properties of the pulsar could be explained if it emitted gravitational waves. In the meanwhile, research had changed directions at FCRAO, turning to interstellar molecular spectroscopy.
In late 1972, ESSCO offered to provide a 14 m diameter antenna, in a protective radome, with a surface of accuracy sufficient for observing at millimeter wavelengths. The telescope was dedicated in 1976 and reached routine operation in 1978. Dickman was the FCRAO manager for a number of years. The radome is shown in Figure B.3. Although studies were made of multiple molecular species, its forte was surveys of CO in our Galaxy, the Milky Way, and in nearby galaxies. The Milky Way surveys were of necessity more limited in extent than those of the Columbia Mini because of the more than 100 times finer resolution in the maps produced, but although they take took longer, more detail was discerned in the final product. The resulting detail allowed for significant new results. For example, it became clear that most of the interstellar molecular gas is in huge, dense clouds, called Giant Molecular Clouds (GMCs). The bulk of star formation in our Galaxy, and presumably other galaxies, occurs in GMCs. FCRAO was also well known for its technical expertise, most notably its array receivers: the 16-element QUARRY and the 32-element SEQUOIA arrays. FCRAO closed in 2006, but its legacy lives on in the careers of many leaders in millimeter astronomy. Its receivers have been repurposed and installed in other telescope projects around the world, most notably, the LMT in Mexico.

Figure B.3 The radome of the FCRAO 14 Meter Telescope at Quabbin Reservoir.
Bell Telephone Laboratories Telescope
Following years of observing at the MWO, the Bell Labs team built their own antenna. It had a unique design that featured an unblocked aperture of 7 m diameter with nothing between the reflecting surface and the sky. The telescope was completed in 1978.5 Work at the facility included studies of isotopic species of interstellar molecules, observations of individual molecular clouds, and surveys6 of CO emission, particularly the 13CO isotopologue. The observing was largely done by Bell Labs staff members, but there was also a visiting observer program. The 7 Meter Telescope is shown in Figure B.4.

Figure B.4 The Bell Labs 7 Meter Telescope with its unblocked aperture. Arno Penzias is in the foreground. (Note: This is not the horn telescope Penzias and Robert Wilson used for their discovery of the cosmic background radiation.)
Millimeter Telescopes Outside the United States
The establishment of millimeter wavelength observatories in the United States was quickly followed abroad. Two large-diameter telescopes were built in the early 1980s which completely outclassed all those in the United States.
Institut de Radio Astronomie Millimétrique
The Institut Radio Astronomie Millimétrique (IRAM) had a somewhat fraught beginning.7 In the 1970s, discussions among the French, German, and British radio astronomers led to an agreement to form the Joint Institute for Millimetre Astronomy. The plan collapsed when the British withdrew. This left the French and German participants to decide what they alone would build, the Germans favoring a large 30 m diameter antenna and the French a millimeter interferometer. When Spain joined the partnership, it became possible to do both. IRAM was founded on 2 August 1979. The 30 Meter Telescope, shown in Figure B.5, was the first of the IRAM facilities to be completed. It is located on Pico Velata near Granada, Spain. Later, an interferometer was completed on the Plateau de Bure, near Grenoble, France. The upgrade of the Plateau de Bure Interferometer, the Northern Extended Millimetre Array (NOEMA) is in operation today, as the premier interferometer for millimeter wavelengths in Europe. IRAM is also a major center for the development of radio astronomy instrumentation and technology.

Figure B.5 The IRAM 30 Meter Telescope on Pico Velata, Spain.
Nobeyama Radio Observatory
Japanese radio astronomy began in the late 1940s with observations of the Sun by researchers at several institutions. A community-wide forum for the promotion of radio astronomy in Japan was organized in December 1969 called the Japan Radio Astronomy Forum. The first millimeter wave telescope was a 6 m antenna installed in 1970 at the Mitaka site of the Tokyo Astronomical Observatory, which later became the National Astronomical Observatory of Japan (NAOJ). In the early 1970s, a plan was developed to construct a 45 m diameter telescope in combination with a five-element millimeter interferometer. These efforts combined at the Nobeyama Radio Observatory (NRO) under the leadership of Masaki Morimoto. The 45 Meter Telescope was to operate at wavelengths from 3 mm to 21 cm. Construction began in 1978 and was completed in 1982. The NRO 45 Meter Telescope continues operations today. It has a novel acoustic spectrometer of 16,000 channels that has been used to discover a number of interstellar molecules. The NAOJ Advanced Technology Center is a major player in the development of millimeter/submillimeter receivers and instrumentation. The 45 Meter Telescope is shown in Figure B.6.

Figure B.6 The NRO 45 Meter Telescope in its mountain valley site.
Onsala Space Observatory
The Onsala Space Observatory (OSO) was founded in 1949 by Olof Rydbeck of Chalmers University. The OSO was dedicated in 1955 and its signature telescope, a 25.6 m diameter antenna, was first operational in 1963. Among the chief programs of research were studies of interstellar CH spectral lines at 9 cm wavelength. These lines were used to study the large-scale structure of the Milky Way. In 1976, a near-twin of the FCRAO millimeter telescope was completed at the OSO. Projects undertaken with this 20 m diameter telescope included a survey of interstellar spectral lines between 72 and 91 GHz emitted from the Orion Nebula and a dust-enshrouded star known as IRC +10216, observations showing that the CO emission from the galaxy M51 follows the spiral arms seen in visible light, and numerous other projects conducted by staff and visiting observers.
Submillimeter Telescopes
One can argue that the history of radio astronomy is a progression from observations at long to ever shorter wavelengths. The field began with Karl Jansky’s discovery of galactic radiation at a wavelength of 14.6 m. In contrast, interstellar CO was discovered at a wavelength of 2.6 mm. The factor of nearly 5,600 in wavelength between these observations was made possible by advances in receiver electronics. As that progression in technology continued, it was inevitable that astronomers would turn to the submillimeter band, that is, wavelengths shorter than 1 mm. Accordingly, starting in the 1980s and up to the time of the writing of this book, the following eight single-dish submillimeter telescopes were built.
James Clerk Maxwell Telescope
In 1983, a partnership between the United Kingdom (UK), the Netherlands, and Canada built the James Clerk Maxwell Telescope (JCMT) on Maunakea. It had a high-accuracy reflecting surface of 15 m diameter capable of submillimeter observations. The main instrument of the JCMT was a pair of receiver arrays, composed of bolometer detectors, called SCUBA. Using SCUBA, observers were able to establish a new class of galaxies called submillimeter galaxies. Today, the telescope has a much more powerful array detector, SCUBA-2, and is operated by a consortium of East Asian countries and UK universities.
Sweden-ESO Submillimetre Telescope
The success of the OSO 20 Meter Telescope’s programs led to the construction of the 15 m Sweden-ESO Submillimetre Telescope (SEST) at ESO’s La Silla site in Chile. Among its successes was a survey of spectral lines from Sgr B2, a strong molecular line source near the center of the Galaxy, over the frequency range 225–250 GHz. Figure B.7 is a photograph of SEST. It was the second millimeter-wavelength telescope built in Chile; a small 60 cm diameter dish had been installed earlier as a test facility by the NAOJ. SEST was decommissioned in 2003. Plans have been announced for moving the SEST antenna to Namibia, where it would serve as a far-south element of the EHT.

Figure B.7 The SEST telescope at ESO, La Silla, Chile.
Caltech Submillimeter Observatory
Bob Leighton built a total of seven aluminum honeycomb sandwich reflector antennas of diameter 10.6 m, Dave Woody overseeing the construction and implementation of the last three. Six went into the OVRO Millimeter Array and the one with the best surface accuracy was used at the Caltech Submillimeter Observatory (CSO), shown in Figure B.8. The CSO was located in the saddle area just below the peak of Maunakea in Hawaii. The measured surface accuracy using astronomical sources is about 20 µm. Submillimeter observing requires very low atmospheric water vapor and even on Maunakea this could be challenging. The CSO was only operated at night to minimize thermal deformations of the reflecting surface. An astrodome reduced the effect of wind. CSO operations began in the 1990s and continued until it was decommissioned in 2015. Over that period the detectors and associated analysis software were steadily improved for use in a variety of research programs. Tom Phillips was the long-time director of the CSO and has written a summary8 of its history and scientific results. Among the more significant are the spectral line surveys, and the discovery that deuterated molecules were much more abundant than anticipated, with even triply deuterated ammonia detectable.9 On 2 March 2021, the Maunakea Management Board approved a plan to deconstruct the CSO and restore its site in 2022. It has been suggested that the CSO be moved to Chile.

Figure B.8 The Caltech Submillimeter Telescope in its astrodome on Maunakea. The JCMT dome is in the upper left.
The Submillimeter Telescope
The push to the submillimeter band also led to the construction of the SMT at the University of Arizona. The SMT is a 10 m diameter telescope housed in an enclosure with large doors that open to the sky. Its construction was completed in 1993 following a fractious debate over the possible impact on the endangered Mt. Graham red squirrel, a situation settled by an act of the US Congress. It was originally built in partnership with the MPIfR and at that time called the Heinrich Hertz Telescope. The SMT is shown in Figure B.9, peering out between the massive open doors of its enclosure.

Figure B.9 The Submillimeter Telescope (SMT) of the University of Arizona. It is one of three telescopes on Mt. Graham, the others being the Large Binocular Telescope and the Vatican Advanced Technology Telescope.
Mt. Fuji Submillimeter Telescope
In 1998, the Japanese, under the leadership of Satoshi Yamamoto, began operation of a submillimeter telescope10 on the summit of Mt. Fuji, at an elevation of 3,725 m. The antenna had a diameter of 1.2 m and was housed in a rotating dome. It was equipped with receivers operating in the 345, 492, and 810 GHz bands and an acoustic-optical spectrometer of 1,024 channels. The telescope is shown in Figure B.10. The principal observing program of this telescope was a survey of emission from neutral carbon atoms in the interstellar medium of the Milky Way.

Figure B.10 The Mt. Fuji Telescope in its radome.
Cologne Observatory for Submillimeter Astronomy
In 1985, Gisbert Winnewisser installed a 3 m diameter antenna in the south tower of the Külm Hotel on the Gornergrat peak above the Swiss village of Zermatt. The telescope’s receivers, covering the frequency range of 210–820 GHz, were used to study interstellar molecular lines. In 1985, the telescope was upgraded with a new mount and reflecting surface. In a partnership with the National Astronomical Observatories of China, the telescope was moved to Tibet in 2010 to become the China-Cologne Observatory for Submillimeter Astronomy. Telescope operation resumed in 2013 following the installation of improved instrumentation.
Atacama Submillimeter Telescope Experiment
Over the two-year period 2002–2004, the NAOJ installed a 10 m diameter telescope at Pampa la Bola, near the future site of ALMA. The site is at an elevation of 4,860 m, slightly lower than the ALMA site. The Atacama Submillimeter Telescope Experiment (ASTE) supports spectroscopic observations at frequencies as high as 350 GHz, or a wavelength as short as 0.87 mm. Figure B.11 shows the telescope with a vehicle for size comparison. ASTE has been a very productive facility since it began operation, with close to 200 science publications and dozens of technical articles. Research has included observations of Galactic molecular clouds and protoplanetary disks, and observations of external galaxies including the Magellanic Clouds.

Figure B.11 The Atacama Submillimeter Telescope Experiment (ASTE) at Pampa la Bola east of the ALMA site on the Llano de Chajnantor.
Atacama Pathfinder Experiment
In a joint venture between MPIfR, OSO, and ESO, a copy of the 12 m diameter ALMA antenna was installed on the Llano de Chajnantor, near ALMA, shown in Figure B.12. The Atacama Pathfinder Experiment (APEX) began operations in 2004. Over 750 scientific articles have resulted from APEX observations, exploring a wide variety of topics. Many of these observations point to further studies with ALMA, but the telescope has proven to be much more than an “ALMA Pathfinder.”

Figure B.12 The Atacama Pathfinder Experiment (APEX) on its Llano de Chajnantor site.
Giant Millimeter Telescopes
Large Millimeter Telescope
A joint project between the United States and Mexico produced the world’s largest millimeter/submillimeter wavelength telescope, the Large Millimeter Telescope (LMT), also known as the Gran Telescopio Millimétrico Alfonso Serrano. The partners are the Instituto Nacional de Astrofísica, Óptica y Electrónica, and the University of Massachusetts, Amherst. Figure B.13 shows the telescope in February 2018, shortly after the 50 m diameter reflecting surface was installed. The telescope operates at wavelengths from 0.85 to 4 mm. An image of the disk surrounding the young star ε Eridani was obtained as an early science result using the AzTEC 1.1 mm continuum camera.11 The LMT has discovered numerous dusty star-forming galaxies at high redshift.12 It is a key element of the EHT.

Figure B.13 The Large Millimeter Telescope (LMT) at an elevation of 4,600 m on Volcán Sierra Negro, the highest mountain in Mexico.
Green Bank Telescope
The Robert C. Byrd Green Bank Telescope (GBT) began operation in 2001. The GBT, shown in Figure B.14, is the world’s largest fully steerable radio telescope. The subreflector and receiver cabin are offset from the telescope’s view of the sky, improving its performance. It has an effective collecting area of 100 m diameter and can support observations at frequencies from 0.1 to 115 GHz. The reflecting surface is made up of 2004 panels supported by motor-driven actuators. On calm winter nights, the actuators can be driven to set the reflecting surface to an accuracy that allows for observing at 3 mm wavelength. The GBT has a large instrumentation suite with the flexibility to support a wide variety of studies: pulsars, atomic hydrogen emission, interstellar molecules, dust emission, radar imaging of solar system objects, and very long baseline interferometry.

Figure B.14 The Robert C. Byrd Green Bank Telescope in the Allegheny Mountains site of the Green Bank Observatory.
Notes
1 The simultaneous discoveries of the first CO outflow sources are reported in Snell, Loren, and Plambeck (Reference Snell, Loren and Plambeck1980) and Rodriguez et al. (Reference Rodriguez1980).
2 For a more complete history of the MWO, see Vanden Bout, Davis, and Loren (Reference Vanden Bout, Davis and Loren2012).
3 See Dame et al. (Reference Dame1987) and Dame and Thaddeus (Reference Dame and Thaddeus2022).
4 Paulikas (Reference Paulikas1976) gives the 1975 annual report for the Aerospace Corp. Millimeter Telescope. See also Dickman, McCutcheon, and Shuter (Reference Dickman, McCutcheon and Shuter1979) and Sargent (Reference Sargent1977).
5 The construction of the 7 Meter Telescope is described in a report published in The Bell System Technical Journal (Chu et al., Reference Chu1978).
6 Stark et al. (Reference Stark, Dickman, Snell and Young1988).
7 The origins of IRAM are given in Open Skies (Kellerman, Bouton, and Brandt, Reference Kellermann, Bouton and Brandt2020). Also, Proposition Commune Pour un Observatoire sur Ondes Millimétriques, February 1975, (NAA-NRAO, MMA, MMA Planning).
8 Phillips (Reference Phillips and Lis2009).
9 Lis et al. (Reference Lis2002).
10 A description of the Mt. Fuji Submillimeter Telescope has been published by Sekimoto et al. (Reference Sekimoto and Butcher2000).
11 See Chavez-Dagostrino et al. (2016).
12 See Berman et al. (Reference Berman2022) and references therein.
Appendix C Lessons Learned
ALMA is by no means the last international science mega-project to be built by astronomers. In radio astronomy alone we have the Next Generation Very Large Array (ngVLA) being developed by NRAO, and the Square Kilometer Array Observatory (SKAO), approved for construction in Australia and South Africa, with operation planned for the late 2020s. At optical wavelengths, we find the Thirty Meter Telescope (TMT), Giant Magellan Telescope (GMT), and Extremely Large Telescope (ELT), all at varying stages of development and construction. It is worth asking if there are any lessons to be learned from the ALMA experience that should be passed along. The authors have asked past ALMA project managers and directors this question. The synthesis that follows comes primarily from the response by Tony Beasley, the ALMA project manager who called for re-baselining the project at the start of his tenure and managed much of the construction. Comments in the list are also based on conversations with and material1 from others with experience in ALMA management.
Carry on in the face of disappointment. The loss of the 25 Meter Project was a bitter defeat, but it led to the MMA. Subsequently, the long delay in funding the MMA was discouraging and frustrating. But consider the alternative: if the NSF had funded the MMA in the early 1990s, NRAO would now be in possession of a facility hardly worth operating. During the delay, the concept grew to become ALMA, something vastly more powerful.
Recognize that politics will have a role in the funding of big projects. This is simply a consequence of democratic government; the taxpayers are entitled to a voice and the support of their representatives is essential to a project’s success.
Do not underestimate the importance of cultural differences. Indifference to cultural differences causes problems that cost time and money to correct, as well as missed opportunities by not recognizing the value that each partner uniquely brings to the collaboration. Goodwill is helpful but not sufficient. Some differences are deeply embedded and cannot be changed. For example, the process of soliciting bids and selecting contractors is very different in Europe, the United States, and Japan, as are the terms and conditions of contracts. The processes can take different amounts of time. Planning is required to take all of this into account. Patience is certainly an asset. Social behaviors, even in the workplace, also differ from culture to culture, and being conscientious of such differences helps to avoid giving offense. Even something seemingly as straightforward as time zones requires extra effort, for example, scheduling worldwide virtual meetings so that the partners take turns attending at three in the morning. Remember that it always sounds worse in writing than you intended it to be. Despite steadily improving technology to connect the world, face-to-face meetings are often necessary. It certainly was the case with ALMA that in-person interactions were better.
Cultural Differences
In February 2003 the Bilateral ALMA Agreement between North America and Europe was signed. The board chair would rotate every two years and Europe would start. In December 2002, I was elected president of ESO Council and thus became the first chairman of the ALMA Board. After two years, Bob Dickman took over, and I was vice-chairman for another year until my term of president of ESO Council ended.
It was a fascinating, but very busy and definitely tumultuous period. What made it special was that Europe and North America were equal partners. The United States was clearly not accustomed to being in that role, but for Europe, being on equal terms with the United States in major scientific undertakings was also unusual. In Europe, submillimeter astronomy was an extension of optical/infrared to longer wavelengths, while in America it was radioastronomy at higher frequencies. Our communities had different backgrounds, but that did not cause the worst headaches. Often practical, but important matters differed: funding cycles, terms of employment, or project management.
Problems took time to solve and too often compromising was seen as losing face. Early on the Board had to choose an ALMA logo. The design (actually adopted) showed some dishes with four stars arranged as the Southern Cross. The American side at first felt it looked too much like ESO’s logo, which also contains that constellation. Then there was the location of the Santiago offices, for which the Vitacura premises of ESO were an obvious option, but only acceptable to the United States if the address would be a different street. These, to Europeans, unimportant issues were resolved but took much time and emotional energy.
There were clashes between personalities, sometimes emotions running very high. No individual, institution, or organization was fully in charge. Persons that had worked hard to realize a millimeter array, saw it develop differently from what they had had in mind. Shaping the collaboration with Chile required diplomacy. We had to deal with cost overruns, deciding on antenna designs, and formalizing the collaboration with Japan into a partnership with yet another culture. The latter made us all minority partners.
Incredibly, in the end every problem was overcome, even the apparently unsolvable ones. It was a great experience working with dedicated persons; I am extremely grateful ALMA came to be and to have been part of it.
Effective professional personnel management is critical. Large projects like ALMA have diverse workforces. The employees have a large range of skills and abilities. Effective personnel management requires a dedicated, professional human resources team if the employees are to be treated fairly. Poor workforce morale can be deadly to a project, or at least imply lower efficiency and potentially slowing progress. Employees should be held to a high standard of behavior if they are to work together effectively. Bad behavior should not be tolerated, even in geniuses.
Expect deliveries to be late. It is inevitable that some, perhaps most, deliveries will be late. Contingency plans should be made with this in mind.
Invest heavily in project management, project engineering, and system engineering. The ALMA project discovered rather late that it needed far more systems engineering than had been planned. NRAO had in past projects left systems engineering to a few talented individuals who mainly conversed with one another and produced modest amounts of documentation. This was inadequate for ALMA. ESO had experience in large project management with the VLT, where they relied on massive amounts of documentation to tie the elements of the project together. Reconciling the two approaches took time. Robust document management software with a top-notch search feature greatly facilitates systems engineering.
Remember that there is no substitute for experience – seek knowledge elsewhere. Construction of a complex facility in a high-elevation remote location will encounter unusual situations and questions. It is a mistake to rely on your own staff to solve every knotty problem. The world is full of experts and there is sure to be one with experience in the most arcane area you encounter. Their advice is worth the cost. ALMA learned high-altitude safety standards from the mining industry and high-altitude medicine from Dr. John B. West, a world expert on the subject.
Make decisions promptly, focusing on the big issues and watching risks. Delay leads to increased costs. It is a mistake to put off a decision until every detail has been examined.
Central control of budget and decision-making is critical. ALMA has a flawed management structure. The central office in Chile responsible for ALMA construction and operations is not a legal entity. All purchases and contracts are made by the ALMA Executives: ESO, NRAO, and NAOJ. It required a director and project manager, who were not only capable directors and managers but possessed the diplomatic skills to secure the support of the Executives for their plans and needs. The structure is cumbersome rather than efficient. That it worked at all, and it did, is a testament to the skill, hard work, and goodwill of those involved.
Accept that some decisions will be made politically. No project the size of ALMA can avoid politics. National interests will be asserted and force some decisions. Learn to live with it.
Recognize that the information volume is too high for good external reviews and reporting. No group of experts, however experienced they may be, can fly in for a three-day project review and grasp all that needs to be understood. High-level reviews are essential to maintain commitments to funding, and as such are unavoidable. One can only hope that a review will focus on the high points and issue an endorsement of the project. Accept that detailed advice might miss the mark.
Funding by the partners should be synchronized. Funding for project construction or operation should arrive from all the partners at the same time. Asynchronous funding requires work arounds that can be difficult to achieve. The delay in the availability of funds from the Japanese government greatly complicated the entrance of Japan into ALMA. It was the goodwill and persistence of all those involved that led to success, but at a significant price in time and effort.
Always keep in mind that, in the end, it’s all about the science! There will be many occasions – dealing with difficult management, administration, human resources, procurement, and funding issues – that will be extremely frustrating and demoralizing. One of senior management’s primary responsibilities is to always exhibit positive leadership to the staff no matter the circumstances, reminding them of the end game, because, “It is all about the science!”
The management of big science projects presents substantial scientific, engineering, informational, and human resource challenges. When multiple institutions are involved, these challenges become even more complex; when the institutions are from various countries and regions of the world, the challenges can be overwhelming. Like people, organizations have their own personalities, habits, and idiosyncrasies. Those participating in ALMA were no exception. NAOJ is a governmental organization, ESO a treaty-based entity, and AUI a private corporation. None of the Executives shared any common legal basis. These very different legal regimes, in turn, gave rise to very different policies and procedures relating to contracting, human resources, scientific staff, and public outreach.
A successful ALMA project required people to work at a level outside their comfort limits and to effect fundamental changes within their organization. To this end, ALMA was most fortunate. While ALMA certainly did not lack for its share of contentious issues, a core of individuals within each ALMA Executive stepped up to develop creative solutions to seemingly insurmountable problems. These individuals developed effective relations with their counterparts, resulting in a reservoir of personal trust and goodwill which would often sustain them during difficult times. Early in ALMA’s existence these small cores of managers recognized that the worst place to thrash out issues were high level meetings, such as the ALMA Board, where protecting an organization’s parochial interests was part of the meeting dynamics. Prior to such meetings, people would meet informally, with their counterparts in the other Executives, to explore where the real boundary conditions were so that options for solutions to difficult issues could be devised prior to the actual meetings. Backchanneling made meetings more efficient and often avoided what otherwise might have been embarrassing situations. Formalized agreements were achieved that could not have been envisioned as even possible had people not challenged themselves and their respective organizations to break out of their normal comfort zones.
Certainly, having to meet a construction schedule helped to spur people into actions and risks that they might otherwise not have taken. But without the right set of people in the right places, ALMA’s eventual success would have been much riskier. In the operations phase of big projects, the need for a core team of creative thinkers willing to go outside of their personal comfort zone and push against their own organization’s normal reflexes continues to be essential. Unfortunately, the need for these qualities often goes unrecognized and under-appreciated.
Note
1 A memorandum, Lessons Learned from ALMA, by E.J. Schreier and J. Webber, September 2010, is an accounting of the reasons behind the cost increases revealed in the re-baselining of the project. It can be found at NAA-PVB, ALMA, ALMA: The Story of a Science Mega-Project. https://science.nrao.edu/about/publications/alma.