Accumulation of vascular smooth muscle cells (VSMC) is a key event in the formation and development of lesions in atherosclerosis. The excessive accumulation of VSMC is due to a combination of directed migration from the media into the intima of the artery accompanied by proliferation and possibly decreased apoptosis. The proliferation and migration of VSMC are associated with vascular change through intimal lesion formation, and these can be induced by growth factors, such as PDGF. PDGF is expressed in various vascular cells such as VSMC, endothelial cells, platelets and macrophages, and platelet-derived growth factor-BB (PDGF-BB) is a more potent inducer of VSMC proliferation and migration than PDGF-AA and -AB(Reference Ross1–Reference Koyama, Hart and Clowes4).
However, the mechanisms related to the PDGF-stimulated proliferation and migration of smooth muscle cells have not yet been fully elucidated. Several studies have shown that PDGF disrupts actin filament assembly and increases the expression of the PDGF-β receptor (PDGFR-β) in injured carotid arteries(Reference Sirois, Simons and Edelman5). PDGF-BB developed intimal thickening and VSMC migration from the media to the intima in a rat model of angioplasty(Reference Jawien, Bowen-Pope and Lindner6). Consistent with the results, it has been shown that a selective PDGF-receptor tyrosine kinase blocker attenuated activation, migration, proliferation and neointimal formation after balloon injury in a swine model(Reference Banai, Wolf and Golomb7). Some studies have also reported that phosphorylations of mitogen-activated protein kinases are related to PDGF-mediated cytoplasmic signalling such as DNA synthesis and the mitogenic process(Reference Bornfeldt, Raines and Nakano8), and the PDGF-induced activation of phospholipase C-γ1 (PLCγ1) and phosphatidylinositol-3 kinase/AKT is implicated in the induction of smooth muscle cell migration(Reference Bornfeldt, Raines and Graves9). Furthermore, PDGF generates reactive oxygen species as a mediator of signal transduction to elicit its mitogenic effect. Thus, PDGF-BB and PDGFR signal transduction are essential for the development and pathogenesis of proliferative vascular disease.
Recently, several compounds from plants have exhibited beneficial effects in the regulation of atherosclerosis, heart diseases and autoimmune disorders. The antioxidant properties of these kinds of compounds have been suggested to explain their beneficial effects. Phytochemicals present in the Leguminosae family of plants have an inhibitory mechanism for PDGF-induced mitogenic signalling in mesangial cells(Reference Venkatesan, Ghosh-Choudhury and Das10). Treatment with phytochemicals derived from soyabean by a biotic elicitor in human aortic VSMC resulted in a significant inhibition of PDGF-mediated cellular proliferation and migration(Reference Kim, Cha and Choi11).
Liquorice, the Glycyrrhiza species (Glycyrrhiza uralensis Fisher), is one of the most frequently utilised plants as a traditional medicine and a natural sweetener since Egyptian, Greek and Roman times, and ancient China(Reference Mae, Kishida and Nishiyama12). Besides antioxidant activity, liquorice root possesses potential beneficial effects against inflammation, viral infection, tumorigenesis, malaria and CVD(Reference Fukai, Marumo and Kaitou13–Reference Lim, Chia and Liong20), as well as peptic ulcers, hepatitis C, and pulmonary and skin diseases(Reference Haraguchi, Ishikawa and Mizutani21, Reference Shibata22).
Glycycoumarin (G. uralensis), glabridin (Glycyrrhiza glabra) and licochalcone A (Glycyrrhiza inflata) have the potential to function as indicators of liquorice(Reference Kondo, Shiba and Yamaji23). As the other bioactive constituents present in liquorice, dehydroglyasperin C (DGC) and dehydroglyasperin D have shown increased PPAR-γ ligand-binding activity and anti-inflammatory activity(Reference Mae, Kishida and Nishiyama12, Reference Kuroda, Mimaki and Sashida24), and isoangustone A has also shown antibacterial effects against methicillin-resistant Staphylococcus aureus (MRSA) strains(Reference Hatano, Shintani and Aga25), suppression of inflammation in renal mesangial cells(Reference Li, Lim and Lee26), and induction of G1 arrest and apoptosis in prostate cancer cells(Reference Seon, Park and Kwon27, Reference Seon, Lim and Choi28).
Although the beneficial effect of liquorice has been postulated in several studies, the protective effect of DGC, a bioactive constituent of liquorice root, on atherosclerosis is not yet known. In the present study, the anti-atherosclerotic effects of DGC were evaluated, and it was found that this compound could inhibit PDGF-stimulated proliferation and migration of human aortic smooth muscle cells (HASMC).
Experimental methods
Preparation of dehydroglyasperin C
Purification of DGC from liquorice root was performed according to the method described previously(Reference Seo, Park and Kim29). Briefly, the n-hexane–ethanol extract of G. uralensis (90 g, 0·9 % yield) was produced by dip extraction with n-hexane–ethanol at a ratio of 9:1 (v/v) of dried and ground roots of G. uralensis (1 kg). A portion of the extract (5·2 g) was subjected to flash column chromatography with silica gel (Macherey-Nagel Kieselgel), eluted by gradient systems of n-hexane–ethyl acetate (5:5, v/v) to obtain twenty fractions. The fractions (0·7 g) showing NAD (P)H:quinone oxidoreductase 1 (NQO1) induction activity were combined and further purified by recrystallisation to recover compound 1 (35 mg). The structure of the compound was elucidated as DGC (Fig. 1) by comparison of the spectral data with an authentic sample.
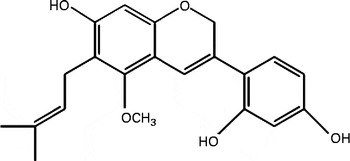
Fig. 1 Molecular structure of dehydroglyasperin C.
Cell culture
HASMC were obtained from Cascade Biologics, Inc. For routine maintenance, cells were grown in Dulbecco's modified Eagle's medium (Gibco) supplemented with 10 % heat-inactivated fetal bovine serum at 37°C in an atmosphere of 5 % CO2–95 % air under saturating humidity and passaged every week (1:4 split ratio) by trypsinisation with 0·25 % trypsin/0·02 % EDTA sodium salt solution (Thermo Fisher Scientific, Inc.).
Cell proliferation assay
The experimental procedures were performed according to previously described methods with some modifications. Briefly, HASMC were trypsinised and plated into ninety-well plates with DMEM at an initial concentration of 1 × 104 cells per well, and incubated in an atmosphere consisting of 5 % CO2 in air at 37°C for 24 h. Then, the medium was removed and replaced by a medium containing various concentrations of the test compounds in serum-free DMEM. The bioassay was terminated on day 1 by removing the medium from the wells, and adding 3-(4,5-dimethyl-thiazol-2-yl)-2,5-diphenyltetrazolium bromide solution (0·5 mg/ml in phenol red-free culture medium). After 2 h, the solution was removed and 200 μl dimethyl sulphoxide was added. After 10 min, absorbance was determined at 540 nm by a microplate reader (Tecan). Cell proliferation of each group was calculated as the relative absorbance of the treatment group to the control.
DNA synthesis assay
Thymidine incorporation experiments were carried out according to a previously described method(Reference Cha, Shi and Yonezawa30). HASMC were cultured until they reached about 40 % confluency. The culture medium was replaced with serum-free medium and incubated for 24 h. The serum-deprived cells were then pre-incubated with DGC for 24 h and stimulated with/without PDGF or with/without DGC for 20 h. After the incubation period, 1 μCi, (37 kBq) of [3H]thymidine was added to the cultures and incubated for 4 h at 37°C. The cells were harvested using a Universal Harvester (Perkin Elmer), and transferred to a GF/C filter (Perkin Elmer). The filter was dried and the level of radioactivity was determined using a microplate scintillation and luminescence counter (TopCount NXT; Perkin Elmer). The values were calculated from absolute counts to a percentage of the control to allow comparison between the experimental groups.
Cell cycle analysis
Cell-cycle progression analysis was measured as described previously(Reference Erl, Weber and Hansson31). HASMC were seeded in six-well plates at 2 × 105 cells/well and incubated until the cell density reached to 80 % confluency; thereafter, the medium was replaced with serum-free medium and incubated for 24 h. The cells were then pre-incubated with DGC for 24 h and stimulated with/without PDGF or with/without DGC for 24 h. HASMC were harvested, resuspended in 70 % ethanol and incubated at − 20°C for 4 h. The fixed cells were washed twice with PBS, and incubated at 37°C for 60 min using a solution containing DNase-free RNase (200 μg/ml). After incubation, the cells were stained with propidium iodide (50 μg/ml) at 4°C for 30 min and subjected to a flow cytometric analysis (Becton Dickinson).
Migration assay
Cell migration was measured with a modified Boyden transwell chamber (twenty-four-well plate) assay as described in our previous study(Reference Cha, Shi and Yonezawa30). Starved cells were trypsinised and cell suspension in a basal medium (500 μl, 5 × 104 cells/well) was seeded in the upper chamber. Then, 760 μl of serum-free medium with or without experimental compounds were added to the lower chamber. After incubation for 24 h, the cells were labelled by the fluorescence dye calcein acetoxymethyl ester (4 μg/ml). The cells that migrated to the lower chamber were measured with a fluorescence microplate reader at an excitation wavelength of 485 nm and an emission wavelength of 530 nm. Each treatment was repeated in three independent transwells.
Western blotting
HASMC were homogenised in pre-cooled radioimmunoprecipitation assay buffer (pH 7·4) containing 50 mm-Tris–Cl, 150 mm-NaCl, 1 mm-EDTA, 1 % Triton-X, 0·5 % sodium deoxycholate and 0·1 % SDS. The homogenates were cleared by centrifugation at 7600 g for 5 min at 4°C, and the supernatants were denatured in sample buffer for 5 min at 95°C. Proteins were separated by electrophoresis on a 10 % SDS–polyacrylamide gel for 1·5 h at 70–150 V and transferred onto nitrocellulose membranes (Amersham Biosciences) for 2 h at 250 mA. The membranes were incubated with antibodies to p-PDGFR-β, PDGFR-β, p-PLCγ1, PLCγ1, p-AKT, AKT, p-extracellular-regulated kinase, extracellular-regulated kinase (ERK), cyclin-dependent kinase (CDK)2, CDK4, cyclin D1, cyclin E and β-actin at dilutions of 1:1000 overnight at 4°C. The bands were detected using a chemiluminescence kit (Pierce). Densitometry analysis was performed with Lab Image software (Scion Corporation).
Angioplasty balloon surgery
Balloon injury in rats was performed as described previously(Reference Clowes, Reidy and Clowes32). All surgical procedures were performed according to the principles of the Kyungpook National University Animal Care and the Guild for the Care and Use of Laboratory Animals. The animals were divided into four groups: negative control (vehicle alone, n 3); balloon injured only (n 3); balloon injured plus DGC (0·5 mg, n 3); balloon injured plus DGC (1 mg, n 3); balloon injured plus DGC (2 mg, n 3). Male Sprague–Dawley rats, 8 weeks old (Daehan Biolink), were anaesthetised using an intraperitoneal injection of Zoletil (1 ml/kg body weight) and Rompun (0·25 ml/kg body weight). The right carotid artery was surgically exposed and injured using a balloon catheter (Edwards Lifescience). After balloon injury, 100 μl of Pluronic gel with or without DGC were applied to the adventitial surface of the injured carotid rat artery.
Tissue preparation and morphological analysis
Carotid arteries were dissected, rinsed with physiological saline and weighed. The tissues were fixed in 10 % buffered formalin (pH 7·6). The fixed tissues were serially sliced at a thickness of 5·0 μm using a microtome (Model RM 2125RT; Leica Microsystems). The sections were stained with Harry's haematoxylin–eosin, and the luminal, neointimal and medial areas of the different sections were quantified with light microscopy and an image analysis program (NIS-Elements F3.00; Nikon Corporation).
Statistical analysis
Data were tested by ANOVA, followed by Duncan's multiple range test, using SPSS software (SPSS, Inc.). The level of significance was set at P <0·05.
Results
Effects of dehydroglyasperin C on human aortic smooth muscle cell proliferation and platelet-derived growth factor-stimulated cycle progression
When HASMC were stimulated by PDGF-BB (20 ng/ml), the number of cells was increased by about 35 % compared with the control group. Treatment of PDGF-stimulated HASMC with DGC (0·1–1 μm) resulted in a significant decrease in cell number, which was not a result of cell death. The ratio of inhibition was 17·3 (sd 3·0), 15·7 (sd 7·8) and 31·0 (sd 6·7) % at 0·1, 0·5 and 1 μm, respectively (Fig. 2(A)). There was no significant change in cell morphology in the cultures treated with PDGF plus DGC (data not shown).
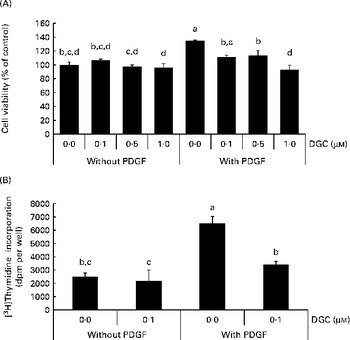
Fig. 2 Effects of dehydroglyasperin C (DGC) on platelet-derived growth factor (PDGF)-induced proliferation of human aortic smooth muscle cells (HASMC). HASMC were treated with or without 1 μm-DGC in the presence or absence of 20 ng/ml PDGF after pre-incubation with or without DGC for 24 h. After incubation for 20–24 h, the cells were processed for the 3-(4,5-dimethyl-thiazol-2-yl)-2,5-diphenyltetrazolium bromide assay (A) or [3H]thymidine incorporation (B) as described in the Experimental methods section. Values are means of three separate independent experiments, with standard deviations represented by vertical bars. a,b,c,dMean values with unlike letters were significantly different (P <0·05).
To confirm the effect of DGC on VSMCC proliferation, we performed DNA synthesis analysis. The effect of DGC on DNA synthesis was assayed by [3H]thymidine incorporation. As shown in Fig. 2(B), incubation of the cells with 20 ng/ml of PDGF led to a significant increase in [3H]thymidine incorporation, which corresponded to approximately a 2·6-fold increase in vehicle-stimulated cells. DGC effectively inhibited the PDGF-induced [3H]thymidine incorporation into DNA. The inhibition percentage of DGC was about 47·9 (sd 4·1) % at 1 μm. These data demonstrate that DGC blocks DNA synthesis in VSMC, resulting in the attenuation of proliferation.
The DNA content was also analysed by propidium iodide staining to investigate the effect of DGC on cell cycle progression in HASMC. PDGF-BB induced an increase in the S phase from 3·4 to 30·5 % compared with the non-stimulated cells. DGC decreased the accumulation at the S phase to 4·6 % with 0·1 μm, 5·9 % with 0·5 μm and 5·7 % with 1 μm in PDGF-treated cells. These results indicate that DGC was effective in arresting PDGF-stimulated cell cycle progression in HASMC (Fig. 3(A) and (B)).
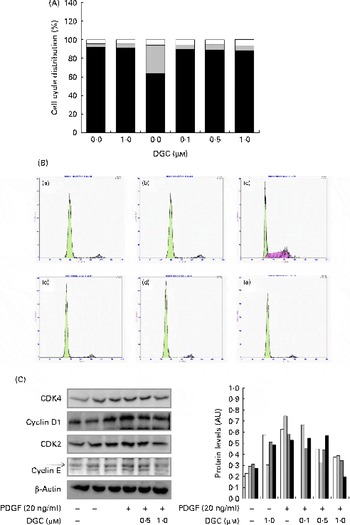
Fig. 3 Effects of dehydroglyasperin C (DGC) on cell cycle progression in human aortic smooth muscle cells (HASMC) stimulated by platelet-derived growth factor (PDGF). HASMC were treated as described in Fig. 2. (A) Representative DNA histograms of propidium iodide fluorescence in cells assessed by flow cytometry are shown. □, G2/M; , S; ■, G0/G1. (B) For evaluation of the expression of proteins related to cell cycle progression, whole-cell extracts (20 μg) were subjected to (C) Western blot analysis for CDK4 (□), cyclin D1 (
), CDK2 (
) or cyclin E (■). AU, arbitrary units. (A colour version of this figure can be found online at http://www.journals.cambridge.org/bjn).
Effects of dehydroglyasperin C on the expression of cell cycle-related proteins
Growth factors increase the expression of cyclin D1 in the G1 phase of the cell cycle to allow entry of the cells into the S phase. Cell cycle progression is tightly regulated through a complex network of positive and negative cell-cycle regulatory molecules such as CDK and cyclins. Along with CDK4, the expression of cyclin E-dependent kinase CDK2 is also essential for the progression of the cells from the G1 to the S phase(Reference Sherr and Roberts33). To investigate whether the inhibition of proliferation by DGC is involved in the regulation of cell cycle-related proteins, we assessed the expression of G1-checkpoint proteins in DGC-treated cycle-arrested cells. As shown in Fig. 3(C), we observed slightly decreased levels of CDK4, cyclin D1, CDK2 and cyclin E in cells incubated with DGC (1 μm). Therefore, these findings suggest that DGC affects the transition of HASMC from the G1 to the S phase, together with its inhibitory effects on HASMC proliferation.
Effects of dehydroglyasperin C on the platelet-derived growth factor-induced signalling pathway
Several phospho-specific antibodies were used to quantify the PDGF-regulated downstream molecules. PDGF-induced activated proteins such as PDGFR-β, PLCγ1 and AKT were detected to determine the inhibitory mechanism of DGC on the signal transduction pathway of PDGF for HASMC proliferation and migration. HASMC were stimulated with PDGF-BB (20 ng/ml) for 15 min, which caused the obvious phosphorylation of PDGFR-β, PLCγ1 and AKT, and the increased phosphorylation was suppressed with various concentrations of DGC (0·1, 0·5 and 1 μm), suggesting that the anti-proliferative effect of DGC was implicated in the early signal transduction by PDGF (Fig. 4(A)–(C)). Since PDGF is capable of inducing the activation of mitogen-activated protein kinases for VSMC proliferation(Reference Bornfeldt, Raines and Graves9), we investigated the effects of DGC on the PDGF signalling pathway by measuring the phosphorylation levels of ERK1/2 with Western blotting (Fig. 4(D)) in HASMC. The results showed that PDGF substantially increased ERK1/2 phosphorylation, whereas cells incubated with DGC at various concentrations (0·1, 0·5 and 1 μm) diminished ERK1/2 phosphorylation in a dose-dependent manner.
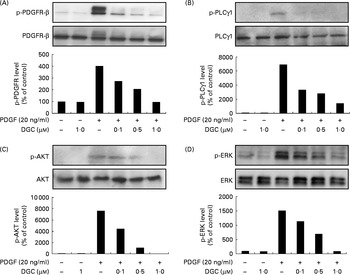
Fig. 4 Effects of dehydroglyasperin C (DGC) on the platelet-derived growth factor (PDGF) signalling pathway. Western blot of the phosphorylation of (A) PDGF receptor-β (PDGFR-β), (B) phospholipase C-γ1 (PLCγ1) and (C) AKT, and (D) the activation of mitogen-activated protein kinases was performed for human aortic smooth muscle cells treated with or without 20 ng/ml of PDGF-BB for 15 min after pre-incubation with DGC for 24 h.
Effects of dehydroglyasperin C on platelet-derived growth factor-stimulated migration of human aortic smooth muscle cells
VSMC migration is affected by the stimulation of PDGFR-β by PDGF(Reference Clowes, Reidy and Clowes32). In order to investigate the effects of DGC on HASMC migration, the cells were treated for 24 h with different concentrations of DGC in the presence or absence of PDGF in a Boyden chamber. The cells migrated to the lower chamber were stained with calcein acetoxymethyl ester, and were photographed with a fluorescence microscope. As shown in Fig. 5, treatment with DGC alone had no significant effect on HASMC migration compared with the vehicle-treated cells. However, PDGF significantly increased the migration up to about 304·4 (sd 29·1) %, while DGC effectively inhibited the PDGF-stimulated cell migration at 1 μm.
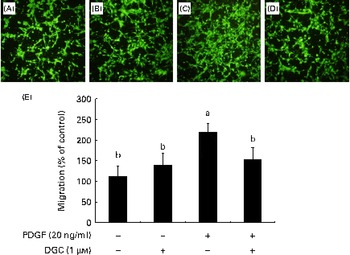
Fig. 5 Effects of dehydroglyasperin C (DGC) on platelet-derived growth factor (PDGF)-induced migration of human aortic smooth muscle cells (HASMC). HASMC were seeded onto the transwell plate with or without 1 μm-DGC in the presence or absence of 20 ng/ml of PDGF-BB for 24 h. The migrated HASMC were analysed with fluorescence calcein acetoxymethyl ester staining. The representative photomicrographs of the migrated cells to the lower chambers after calcein acetoxymethyl ester staining are shown as follows: (A) control; (B) DGC (1 μm); (C) PDGF; (D) PDGF+DGC (1 μm). The percentage of migrated cells relative to the control is shown in the bar graph (E). Values are means of three separate independent experiments, with standard deviations represented by vertical bars. a,bMean values with unlike letters were significantly different (P <0·05). (A colour version of this figure can be found online at http://www.journals.cambridge.org/bjn).
Effects of dehydroglyasperin C on the rearrangement of actin filaments induced by platelet-derived growth factor
Regardless of the cell type, since effective reorganisation of the actin cytoskeleton is absolutely important for migration(Reference dos Remedios, Chhabra and Kekic34), we attempted to determine the effects of DGC on the PDGF-induced rearrangement of actin filaments (Fig. 6). Indeed, we observed that HASMC underwent a reorganisation of actin after the addition of PDGF, and DGC alone did not show any change in the actin microfilaments of HASMC. On the contrary, incubation with DGC (1 μm) in the presence of PDGF blocked the loss of microfilaments, and the appearance of the actin filaments looked similar in the cells treated with either the vehicle or DGC alone.
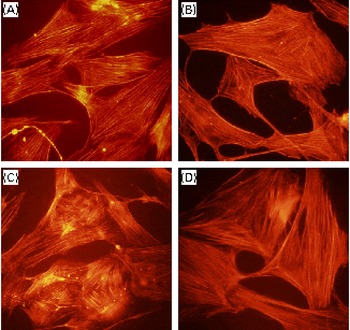
Fig. 6 Effects of dehydroglyasperin C (DGC) on platelet-derived growth factor (PDGF)-stimulated cytoskeletal reorganisation. The cells grown on a coverslip were treated with or without 1 μm-DGC in the presence or absence of 20 ng/ml of PDGF-BB for 24 h. The cells were fixed, and the actin filaments were stained with rhodamine phalloidine and photographed using a fluorescent microscope. (A) Control; (B) DGC (1 μm); (C) PDGF; (D) PDGF+DGC (1 μm). Magnification 200 × . (A colour version of this figure can be found online at http://www.journals.cambridge.org/bjn).
Dehydroglyasperin C inhibits neointimal formation in an animal model of angioplasty restenosis
DGC induced a dose-dependent reduction in intimal hyperplasia compared with the untreated group (Fig. 7(A) and (B)). Treatment with DGC inhibited the balloon injury-induced decrease in the luminal area (balloon injured only, 0·146 (sd 0·001); balloon injured plus DGC (0·5 mg), 0·219 (sd 0·005); balloon injured plus DGC (1 mg), 0·261 (sd 0·006); balloon injured plus DGC (2 mg), 0·285 (sd 0·024)). As shown in Fig. 7(B), a significant reduction in the luminal area by DGC was related to the intima:media area ratio. In addition to the reduction in the medial area (about 18 %), the DGC-treated group also showed a reduced neointima:media area ratio than the vehicle-loaded Pluronic gel group in a dose-dependent manner (balloon injured plus DGC (0·5 mg), approximately 38 %; balloon injured plus DGC (1 mg), approximately 68 %; balloon injured plus DGC (2 mg), approximately 84 %).
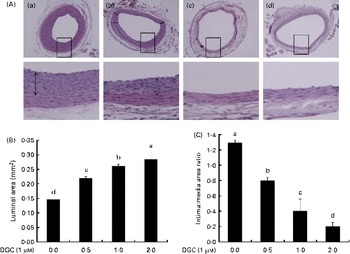
Fig. 7 Effects of dehydroglyasperin C (DGC) on rat carotid artery morphology after balloon injury. DGC (0·5 and 1 mg) diminished the formation of intimal smooth muscle cells on the 16th day after balloon catheter injury. (A) Control (a), 0·5 mg DGC (b), 1 mg DGC (c) and 2 mg DGC (d). Magnification 100 × and 400 × . (B) Luminal area and (C) intima:media area ratios of the four groups (n 4 per group). a,b,c,dMean values with unlike letters were significantly different (P <0·05). (A colour version of this figure can be found online at http://www.journals.cambridge.org/bjn).
Discussion
VSMC are major cells present in arterial structures, and play a very important role in the maintenance of normal vascular structures and functions. VSMC are normally quiescent, at low proliferative indices, and remain in the G0/G1 phase of the cell cycle(Reference Xiong, Hannon and Zhang35). The growth of VSMC is an indicator for the formation and development of atherosclerosis through proliferative responses and promoting the expression of other growth factors in VSMC(Reference Ferns, Raines and Sprugel36, Reference Newby and Zaltsman37).
Several studies have shown that liquorice-derived compounds have beneficial effects on atherosclerotic processes including the adhesion and migration of leucocytes(Reference Chang, Chen and Kuo19), development of visceral obesity, regulation of HDL levels(Reference Eu, Lim and Ton18) and lipidaemia(Reference Lim, Chia and Liong20), and vascular growth factor-induced proliferation of VSMC(Reference Park, Lim and Lee38). In addition to vascular protective effects, liquorice is also known to possess antioxidant activity(Reference Chin, Jung and Liu16).
Although the constituents of liquorice are desirable candidates acting as anti-atherosclerotic agents, little is known regarding their effects on PDGF-induced proliferation and migration of VSMC. In the present study, we tested the inhibitory effects of DGC on PDGF-induced proliferation and migration of HASMC, and attempted to explain its associated mechanisms. PDGF was used as a positive control to evaluate the effect of DGC on the proliferation of VSMC. We provide the first evidence that DGC inhibits PDGF-stimulated proliferation and DNA synthesis without induced apoptosis.
pRb, CDK, cyclins, CDK inhibitor proteins, p21cip1 and p27kip1, and tumour-suppressor proteins including p53(Reference Sherr and Roberts33, Reference Braun-Dullaeus, Mann and Sedding39, Reference Sherr40) are implicated in controlling the cell cycle for the G1, S, G2 and M phases. While normal VSMC maintain the G0 phase for a quiescent state, an increase in the accumulation of VSMC in the intima and media is related to excessive proliferation and diminished VSMC death. CDK2 and CDK4, important mediators in modulating the G0/G1- to S-phase progression of the cell cycle and function by building complexes with cyclin E and cyclin D1, respectively, increase the activation of pRb and release transcription factors, resulting in the promotion of DNA synthesis(Reference Sherr and Roberts33, Reference Sherr40–Reference Martin, Odajima and Hunt42). On that account, the methods controlling proliferation and reducing apoptosis in abnormal VSMC have been developed as a new therapy(Reference Guevara, Chen and Chan43). In the present study, DGC restrained the PDGF-induced proliferation at a concentration of 1 μm, which may be attributable to the arrest of the G0/G1–S phase by reducing the expression of cyclin E/CDK2 and cyclin D1/CDK4. This reduced expression suggests that PDGF-stimulated expression of cell-cycle regulatory proteins is necessary for this inhibitory activity of DGC to alleviate the abnormal proliferation of VSMC.
PDGFR-β was expressed in the VSMC of atherosclerotic lesions(Reference Wilcox, Smith and Williams44). It has been reported that the activated PDGFR in PDGF-BB-stimulated VSMC produces H2O2, resulting in the phosphorylation of the mitogen-activated protein kinases ERK1/2(Reference Sundaresan, Yu and Ferrans45) and PLCγ1(Reference Sundaresan, Yu and Ferrans45–Reference Choi, Lee and Kim47). In addition to reactive oxygen species, PDGFR-β phosphorylated by PDGF interacts with PLCγ, Ras guanine 5′-triphosphatase-activating protein, phosphatidylinositol-3 kinase, tyrosine phosphatase SH2 domain-containing tyrosine phosphatase 2 (SHP-2) and members of the signal transducers and activators of the transcription family that are present in the cytoplasm(Reference Heldin and Westermark48).
Recently, DGC among the bioactive compounds from liquorice root has been shown to induce the expression of detoxifying enzymes through the transcription factor Nrf2 in hepatoma cells(Reference Seo, Park and Kim29), and exhibited antioxidant activity against 2,2′-azino-bis(3-ethylbenzothiazoline-6-sulfonic acid) (ABTS+) radicals(Reference Lee, Kim and Kim49). In our preliminary study, DGC showed an antioxidant property in rat tissues (data not shown), which has been proved by a significant inhibition of lipid peroxidation, increased proliferation of oestrogen receptor (ER)-positive MCF-7 cells, elevated oestrogenic activity on yeast-based ER activity and higher affinity to ER-β than to ER-α. The animal study performed by Hsieh et al. (Reference Hsieh, Liu and Hsu50) also demonstrated that an α-tocopherol derivative inhibited PDGF-stimulated neointimal formation after angioplasty through the inhibition of reactive oxygen species-mediated PLCγ1–PKCδ and Janus kinase 2 (JAK2)–signal transducers and activators of transcription 3 activation, which cause the cell cycle arrest of the G2/M phase. Furthermore, the constituents derived from the plant containing antioxidant activities were elucidated to have anti-proliferative properties in vascular cells induced by PDGF(Reference Kim, Cha and Choi11). Considering the correlation among atherosclerosis, the oxidation process and the influence of natural oestrogen derived from plants(Reference Sosroseno, Bird and Seymour51–Reference Kim, Suh and Kim53), there is a possibility that DGC may control vascular growth factor-mediated morphological changes in the blood vessels. As expected, the present results showed that DGC regulated the proliferation of vascular cells by reducing Akt and PLCγ1 in PDGF-treated HASMC.
ERK1/2 controls the PDGF-induced proliferation of VSMC through the regulation of the cell cycle modulators cyclin D1 and p27kip1(Reference Martin, Odajima and Hunt42, Reference Wei, Krasinski and Kearney54–Reference Castro, Diez-Juan and Cortes56). Mitogenic stimulation activates the kinase MEK1/2 and induces the subsequent phosphorylation of ERK1/2 that activates downstream transcription factors such as Elk-1(Reference Yang, Madinova and Kozai57), which is involved in DNA synthesis in VSMC(Reference Adam, Borer and Williams58). Consistent with previous studies, the present data showed that DGC significantly decreased the PDGF-induced phosphorylation of ERK1/2.
VSMC migration from the media to the intima in the blood vessels leads to intimal thickening. In this process, PDGF is an important migratory factor for VSMC during neointimal formation(Reference Jalvy, Renault and Leen59). Although the mechanisms of PDGF-stimulated migration have not been fully understood so far, there are several interesting studies. For instance, PDGF-BB facilitated intimal hyperplasia through the media-to-intima migration of VSMC in an animal model(Reference Jawien, Bowen-Pope and Lindner6), and the blocking of PDGF receptor signalling markedly retarded VSMC growth and neointimal formation by injury(Reference Banai, Wolf and Golomb7). In addition, it is known that PDGF disturbs actin filament assembly and gives rise to an excessive increase in PDGFR expression after angioplasty(Reference Sirois, Simons and Edelman5).
Taken together, the present study demonstrates that DGC has the ability to inhibit not only PDGF-stimulated proliferation of VSMC, which is attributable to interference with the cell cycle, but also VSMC migration. The possible mechanisms of DGC responsible for inhibiting the proliferation of VSMC are related to the inactivation of PDGFR-β, PLCγ1, AKT and ERK. The results of the present study will be helpful in better understanding the mechanism of the pharmacological activity of DGC in the treatment of atherosclerosis.
Acknowledgements
This study was supported by the Basic Science Research Program through the National Research Foundation of Korea (NRF) funded by the Ministry of Education, Science and Technology (2010-0027204). J.-S. K. and J.-T. W. planned the study. H. J. K. and B.-Y. C. performed the proliferation, Western blotting, migration and cytoskeletal reorganisation assay. H. J. K., I. S. P. and J. S. L. carried out the angioplasty balloon surgery. H. J. K., B.-Y. C. and J.-S. K. wrote the paper. The authors declared no conflict of interest.