1. Introduction
This paper tells the story of a most interesting form of sea ice, which many people may never have heard of. Platelet ice, as introduced in a schematic diagram in Figure 1 and in a collection of photos in Figure 2, is a very peculiar and unusual type of sea ice, which is most commonly found along the coast of Antarctica (Langhorne and others, Reference Langhorne2015). Its presence results from an interaction between the ocean, ice shelves (floating extensions of the continental ice sheet) and immobile, landfast sea ice attached to the latter (Fraser and others, Reference Fraser, Massom, Michael, Galton-Fenzi and Lieser2012). Its appearance has fascinated researchers ever since its first documented observation in McMurdo Sound during the Discovery Expedition of 1904–1905 (Hodgson, Reference Hodgson1907). In contrast to regular sea ice, which initially forms and grows at the ocean surface by heat conduction to the atmosphere (Stefan, Reference Stefan1891), platelet ice originates from supercooling of sea water deeper in the water column, inside the ice-shelf cavity. There, basal ablation of the ice shelf cools and freshens the surrounding ocean water, which then becomes more buoyant, and ascends towards the surface. The accompanying pressure relief can cause this water to have a potential temperature below the in situ freezing point, a condition which is called in situ supercooling (Foldvik and Kvinge, Reference Foldvik and Kvinge1974).
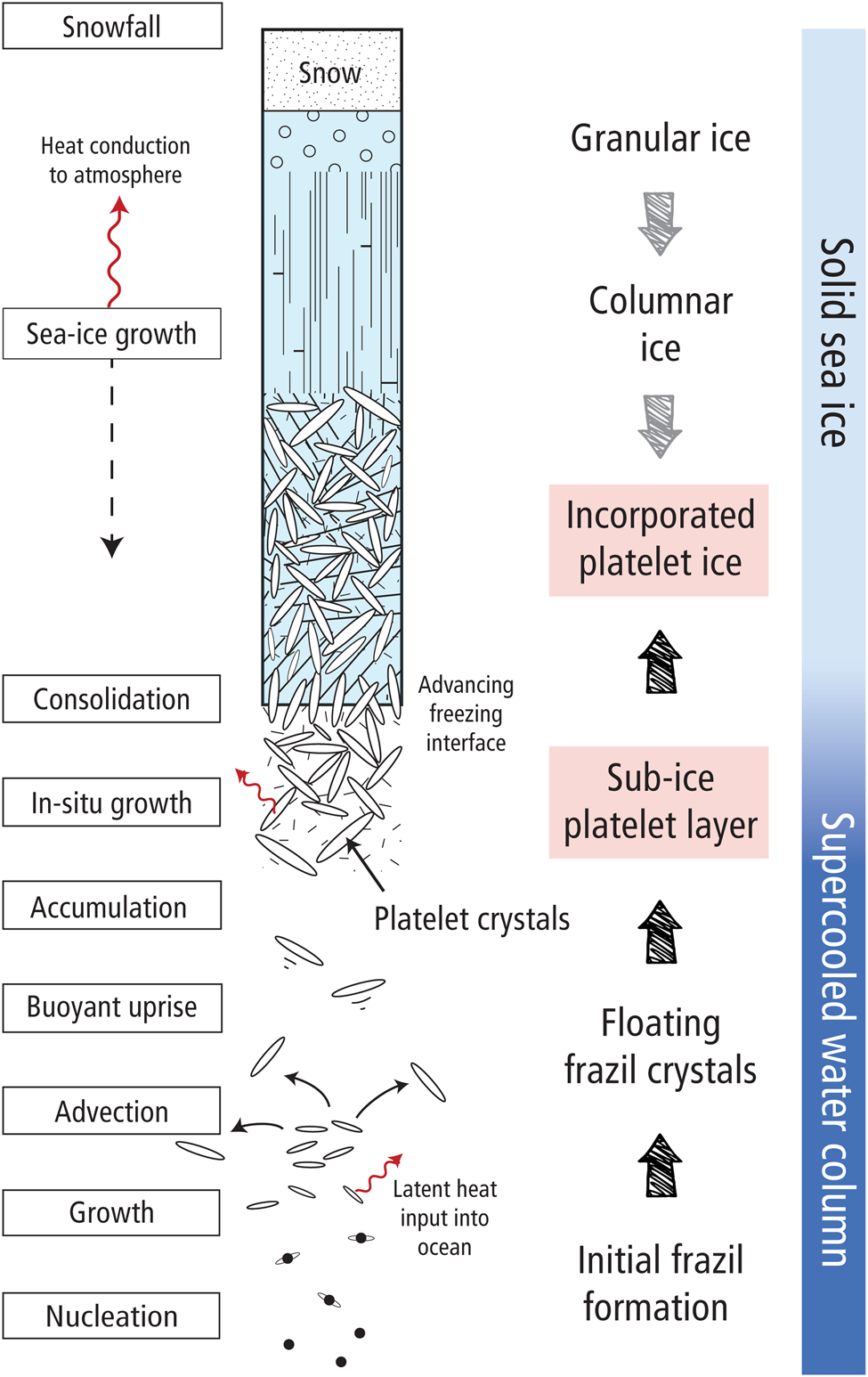
Fig. 1. Schematic illustration of an Antarctic sea-ice cover close to an ice shelf that was modified by platelet ice (not to scale). Initially, a granular ice cover forms from ice crystals at the sea surface, and grows to form columnar ice by heat transport to the atmosphere. At the same time, suspended frazil crystals also form in supercooled water at depth from a different process, which then are advected by currents, and eventually rise to the surface. The growing crystals accumulate into a porous network of disordered platelets, which constitute the sub-ice platelet layer. Throughout winter, the freezing interface advances into this layer, leading to a progressing consolidation and eventually forming incorporated platelet ice.
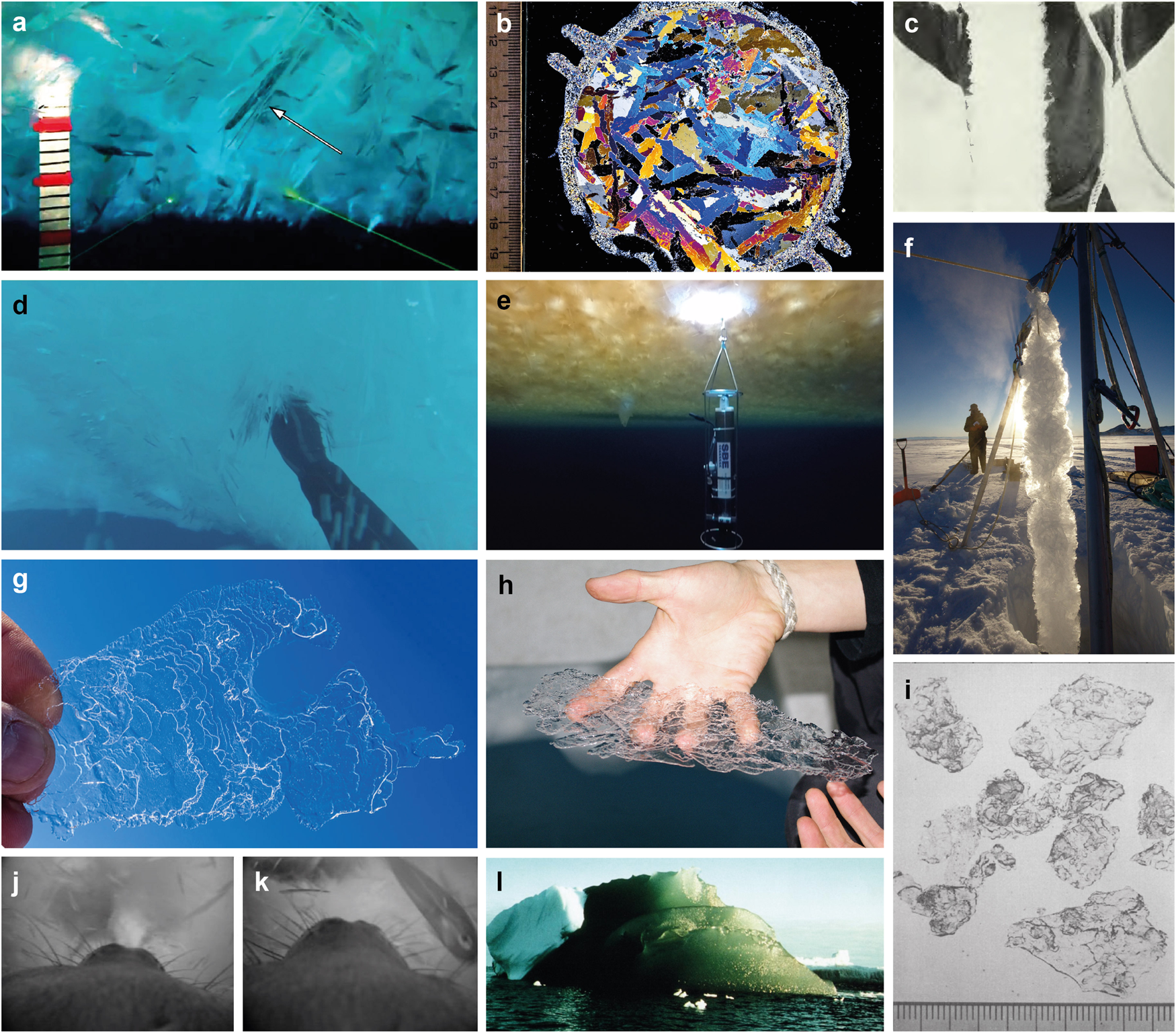
Fig. 2. Collage of platelet ice and associated ice types discussed in this paper: (a) view of sub-ice platelet layer (SIPL) by a camera with laser and ruler (Mahoney and others, Reference Mahoney2011); (b) horizontal thin section showing incorporated platelet ice in Atka Bay; (c) the earliest published photo of ice on a rope (Wright and Priestley, Reference Wright and Priestley1922); (d) diver's hand reaching into SIPL in Atka Bay (Philipp Fischer, AWI); (e) oceanographic instrument lowered through SIPL in McMurdo Sound (2016 K066 team); (f) ice on rope in McMurdo Sound (Mahoney and others, Reference Mahoney2011); (g, h) individual platelet crystals retrieved in Atka Bay (Mario Hoppmann) and McMurdo Sound (Craig Stevens, NIWA); (i) frazil crystals fished by net trawl in the Weddell Sea (Dieckmann and others, Reference Dieckmann, Rohardt, Hellmer and Kipfstuhl1986); (j, k) fish escaping the SIPL after a seal blast (Davis and others, Reference Davis1999); (l) green iceberg composed of marine ice (Kipfstuhl and others, Reference Kipfstuhl, Dieckmann, Oerter, Hellmer and Graf1992). The most extensive collection of video material so far can be found on the webpages and video channel of the McMurdo Oceanographic Observatory (MOO) that has been installed on the shallow seafloor in McMurdo Sound in late 2017 (Cziko, Reference Cziko2019).
The first study to hypothesise that platelet ice forms through heat transport into the ocean was performed by Wright and Priestley (Reference Wright and Priestley1922). Reporting on observations made during the British Terra Nova Antarctic Expedition 1910–1913, they were also the first to see the potential significance of this phenomenon, stating that ‘[…] later in the winter the sea ice grows to its great thickness of 8 and 9 feet largely by the deposition of frazil crystalsFootnote 1 from below’. Although a number of processes exist which may lead to supercooling conditions in polar oceans (Martin, Reference Martin1981; Jeffries and others, Reference Jeffries1995; Mager and others, Reference Mager, Smith, Kempema, Thomson and Leonard2013), only specific patterns of thermohaline convection beneath an ice shelf are able to generate and maintain supercooling of sufficient strength, spatial extent and permanency to lead to substantial platelet-ice formation (Foldvik and Kvinge, Reference Foldvik, Kvinge and Dunbar1977, see Section 3 of this paper). Although the early insight by Wright and Priestley (Reference Wright and Priestley1922) was accurate, they did not fully grasp the profound consequences this phenomenon has on the local cryosphere and biosphere. More than 100 years and many publications later, it is still incredibly difficult even for scientists from the relevant disciplines to fully appreciate, let alone understand, all the effects and implications that accompany platelet ice.
One of the main reasons is that, although researchers from diverse backgrounds have looked at this phenomenon from various angles, the limited number of interdisciplinary studies has led to a highly ambiguous terminology (see Section 2). As a result, a comprehensive picture of platelet-ice properties as well as its geographical distribution has not yet been established, and a solid understanding of its role in both the local and global contexts is lacking. This is a significant gap in our otherwise emerging understanding of ice–ocean interaction, which this paper aims to fill. In the Conclusions (Section 9), we present a flow chart (Fig. 6) that attempts to illustrate all the involved processes, implications and interactions in one comprehensive figure, and that can be considered a graphical summary of this paper.
The term ‘platelet ice’ either refers to (1) loose accretions of individual ice crystals directly underneath drifting (Eicken and Lange, Reference Eicken and Lange1989) and landfast sea ice (e.g. Moretskii, Reference Moretskii1965; Dayton and others, Reference Dayton, Robilliard and Devries1969; Cherepanov and Kozlovskiy, Reference Cherepanov and Kozlovskiy1972; Gow and others, Reference Gow, Ackley, Weeks and Govoni1982; Arrigo and others, Reference Arrigo1995; Robinson and others, Reference Robinson, Williams, Stevens, Langhorne and Haskell2014; Hoppmann and others, Reference Hoppmann2015b), or (2) the part of a solid sea-ice cover which has such crystals incorporated in its usually columnar fabric (e.g. Jeffries and Weeks, Reference Jeffries and Weeks1992; Jeffries and others, Reference Jeffries, Weeks, Shaw and Morris1993; Gow and others, Reference Gow, Ackley, Govoni and Weeks1998; Tison and others, Reference Tison1998). Refer to Section 2 for the exact definitions, and to Figure 2 for a collage of relevant images. Both forms come with its own specific consequences, which will all be described in the following. The occurrence of platelet ice in either its loose or consolidated form is limited to the Antarctic coast, in regions where cold-cavity ice shelves are present (Fig. 4, updated from Langhorne and others, Reference Langhorne2015), and mostly associated with landfast sea ice. Although similar observations have sporadically been made in the Arctic (Jeffries and others, Reference Jeffries1995; Kirillov and others, Reference Kirillov2018), these were the result of different processes, such as refreezing melt ponds or the sudden release of snow meltwater.
Where present, platelet ice interacts with its surroundings in many ways (Fig. 6). Individual aspects of platelet ice and their impact on the local environment have been addressed in a number of studies from many different scientific disciplines, such as glaciology (e.g. Warren and others, Reference Warren1993; Smith and others, Reference Smith2001; Craven and others, Reference Craven, Allison, Fricker and Warner2009), oceanography (e.g. Leonard and others, Reference Leonard2011; Mahoney and others, Reference Mahoney2011), geophysics (e.g. Hunkeler and others, Reference Hunkeler, Hoppmann, Hendricks, Kalscheuer and Gerdes2016b), remote sensing (e.g. Price and others, Reference Price2014), numerical modelling (e.g. Dempsey and others, Reference Dempsey2010; Wongpan and others, Reference Wongpan, Langhorne, Dempsey, Hahn-Woernle and Sun2015), ecology (e.g. Arrigo and others, Reference Arrigo1995; Günther and Dieckmann, Reference Günther and Dieckmann1999; Vacchi and others, Reference Vacchi2012) and biogeochemistry (e.g. Günther and others, Reference Günther, Gleitz and Dieckmann1999b; Carnat and others, Reference Carnat2014), underlining its multi-faceted role. At the same time, the detection of its presence is very challenging due to its difficult-to-access location within or beneath a sea-ice cover. In particular, currently available observational techniques (Section 4) limit the determination of its extent, as well as its physical and biogeochemical properties.
The major aim of this review is to provide a comprehensive overview of the properties and implications of this extraordinary sea-ice type. In doing so, we try to consolidate our present understanding of this diverse topic in a way understandable for all relevant disciplines, hoping to facilitate and stimulate much needed multidisciplinary research.
Due to the challenges associated with the ambiguous terminology and the historical nature of some of the observations, a standard literature search did not yield a satisfactory overview. Instead, we scanned the reference lists of numerous papers from various disciplines, trying to identify as many relevant papers as possible. Although likely still not complete, one of the main outcomes of the current paper is the most comprehensive and extensive list of references dealing with the topic of platelet ice. In the following, we summarise what we consider the most relevant information from this collection.
We start with a clarification of the terminology (Section 2) which, on the initiative of the authors, will be included in the next update of the WMO Sea-Ice Nomenclature compiled by the JCOMM Expert Team on Sea Ice (2015). After reviewing in detail the formation process (Section 3), we introduce the most common observational and numerical techniques to investigate platelet ice (Section 4), before we build upon the work of Langhorne and others (Reference Langhorne2015) to give an overview of all published observations of platelet ice around Antarctica we are aware of to date (Section 5). After an extended discussion of the properties of platelet ice (Section 6), and how it interacts with its surroundings (Section 7), we speculate on the fate of platelet ice in a warming world (Section 8). We conclude this work with a synthesis of our findings, highlighting the major gaps and challenges that need to be addressed in the future (Section 9).
2. Terminology
The terminology related to ice formation and growth in supercooled water is extremely ambiguous, and to an extent, even chaotic. A lot of confusion arises from the fact that different disciplines use different terms for essentially the same type of ice, mainly as a result of their different methods and approaches. In addition to the use of entirely different terms such as ‘underwater ice’ (Moretskii, Reference Moretskii1965; Cherepanov and Kozlovskiy, Reference Cherepanov and Kozlovskiy1972, Reference Cherepanov and Kozlovskiy1973; Kipfstuhl, Reference Kipfstuhl1991), there is particular inconsistency in the ways that the terms ‘frazil ice’, ‘platelet ice’, ‘anchor ice’ and even ‘marine ice’ are used in the literature, often interchangeably, as pointed out by Smith and others (Reference Smith, Langhorne, Trodahl, Haskell and Cole1999) and Mager and others (Reference Mager, Smith, Kempema, Thomson and Leonard2013). To make it even more confusing, authors sometimes used different expressions for the same ice type in the same publication. As noted by Smith and others (Reference Smith, Langhorne, Trodahl, Haskell and Cole1999), the term ‘platelet ice’ is often used at the same time to describe individual platelet crystals, their accumulations in layers and the structural component of solid sea ice modified by the incorporation of these crystals. Due to the profoundly different impacts and roles of these manifestations for the local systems it is urgently necessary to make a proper distinction.
To improve the communication and mutual understanding of the many involved disciplines, it is crucial to determine and adopt a unified nomenclature. Based on the most recently published literature (Mager and others, Reference Mager, Smith, Kempema, Thomson and Leonard2013; Langhorne and others, Reference Langhorne2015; Smith and others, Reference Smith2015; Hunkeler and others, Reference Hunkeler, Hoppmann, Hendricks, Kalscheuer and Gerdes2016b), we recommend using the terms ‘sub-ice platelet layer’ for loose accretions of ice crystals under sea ice, ‘incorporated platelet ice’ for solid sea ice with platelet (ice) crystals incorporated in its fabric and use ‘platelet ice’ as a generic term only when a further specification is not deemed necessary. Small, individual ice crystals suspended in the water column continue to be referred to as ‘frazil’ in accordance with the existing definition from the JCOMM Expert Team on Sea Ice (2015), a term which has been used by the sea-ice community for decades. A future community discussion on possible changes to this rather general and ambiguous term would be useful. Instead, we suggest the term ‘platelet crystals’ for the individual crystals within either a sub-ice platelet layer or incorporated platelet ice (see Fig. 1 and Table 1).
Table 1. Definitions of the terminology used in this paper

On the authors' initiative, the definitions of ‘platelet ice’, ‘sub-ice platelet layer’, ‘incorporated platelet ice’ and ‘platelet crystals’ will be included in the updated version of the WMO Sea-Ice Nomenclature (JCOMM Expert Team on Sea Ice, 2015).
As with every general definition, especially in the realm of sea ice, there are still some ambiguities and issues that remain unaccounted for: due to the lack of significant platelet-ice observations in the Arctic, we decided to introduce here a geographical limitation to the Antarctic. This may need to be revised in the future. Because of the impracticability of a distinction based on formation history, shape or size of individual crystals, another potential shortcoming is the lack of a distinction in the definition between frazil and a mobile sub-ice platelet layer. These shortcomings might (or should) change in the future. At this point however, we urge any authors to be particularly careful with these terms and stick to the proposed nomenclature especially for consistency reasons, until new knowledge has been obtained and any adjustment becomes necessary.
3. Platelet-ice formation in Antarctica
The usual process of sea-ice formation takes place at the ocean surface, when the colder atmosphere extracts heat from the ocean surface (Stefan, Reference Stefan1891). In the polar oceans, sea ice may also form deeper in the water column, under conditions where the water becomes ‘in situ supercooled’, that is, colder than its freezing-point temperature (Martin, Reference Martin1981; Daly, Reference Daly1994; Mager and others, Reference Mager, Smith, Kempema, Thomson and Leonard2013). This process may occur in surface layers of leads and polynyas, at the interface between two fluid layers with different salinities, and, as reviewed in this paper, on a much larger scale in the water column adjacent to or below ice shelves (Martin, Reference Martin1981). Approximately half of Antarctica's coastline is fringed by ice shelves (Fretwell and others, Reference Fretwell2013). These are large, floating sheets of freshwater ice which extend hundreds of metres into the water column. Melting at the base of these ice shelves through contact with relatively warm water masses is a major contributor to Antarctica's ice-sheet mass budget (Pritchard and others, Reference Pritchard2012; Depoorter and others, Reference Depoorter2013; Rignot and others, Reference Rignot, Jacobs, Mouginot and Scheuchl2013) and is a prerequisite for platelet-ice formation. In the following subsections, we will give a brief introduction to the physical processes involved in its formation. Additionally, a simplified scheme illustrating all the relevant components of this system, as well as their interlinkages, is presented in Fig. 3.

Fig. 3. Sketch of the ice pump process in an ice-shelf cavity. High Salinity Shelf Water (HSSW) enters the cavity and melts the base of the ice shelf. Basal melting leads to the formation of very cold, less saline Ice Shelf Water (ISW). The ISW rises, and the plume becomes in situ supercooled, as the freezing point depends on the pressure. Under these conditions, frazil crystals form in the water column. The crystals float upwards, where they may grow further. If they accumulate beneath the ice shelf, they are termed ‘marine ice’ (see Section 3.5), beneath the sea ice they are referred to as ‘sub-ice platelet layer’. If they grow on parts of the shallow seafloor (not shown), they become ‘anchor ice’ (see Section 3.8). Graphic modified from Hughes (Reference Hughes2013).
3.1 Melting or dissolving?
The ocean-driven, solid-to-liquid phase transition at the base of Antarctic ice shelves is dominated by either one of two different thermodynamic ablation processes: dissolving or melting (Woods, Reference Woods1992). At ocean temperatures higher than the in situ sea-water freezing temperature (~−2.5°C at 800 m) and lower than the in situ freshwater ice melting point, ice is dissolved by the saline solute. The rate of ice loss is controlled by the supply of salt and heat to the ice–ocean interface, a process which is dominant at the deep grounding line of cold-cavity ice shelves. When the ocean is warmer than ~−0.5°C at the surface (Wells and Worster, Reference Wells and Worster2011), melting takes place; the ablation rate is controlled by heat transport to the ice–ocean interface only (e.g. at ice-shelf fronts in summer surface water). As the molecular diffusivity of salt is much smaller than the diffusivity of heat (mass diffusivity, D ~ 5 × 10−10 m2 s−1, while heat diffusivity, κ ~ 10−7 m2 s−1), ablation by melting is of order (κ/D)3/4 ~ 50 times faster than by dissolving in environments of low turbulent kinetic energy (Woods, Reference Woods1992; Kerr, Reference Kerr1994; Notz and others, Reference Notz2003; Wells and Worster, Reference Wells and Worster2011). However, throughout this paper we will omit the distinction between melting and dissolving. Instead, we use the terms ‘melting’ and ‘ablation’ interchangeably when referring to the phase change of ice to water.
3.2 How does Ice Shelf Water form?
Melting at the base of ice shelves can form Ice Shelf Water (ISW) (Jacobs and others, Reference Jacobs, Fairbanks and Horibe1985), a water mass characterised by a potential temperature below the surface freezing point (called potential supercooling; Jacobs, Reference Jacobs2004). ISW is part of a thermohaline convection mechanism under and in front of ice shelves (Foldvik and Kvinge, Reference Foldvik, Kvinge and Dunbar1977; MacAyeal, Reference MacAyeal1984), that is also referred to as ‘ice pump’ (Section 3.5, Lewis and Perkin, Reference Lewis and Perkin1986). Its presence is a necessary but not sufficient prerequisite for the formation of platelet ice (Mahoney and others, Reference Mahoney2011).
3.3 How does High Salinity Shelf Water form?
Growing sea ice produces cold and saline surface water due to salt rejection during freezing. This process is enhanced in coastal polynyas, where an extensive air–water interface is maintained by seaward katabatic winds continuously driving the new sea ice offshore (Massom and others, Reference Massom, Harris, Michael and Potter1998; Maqueda and others, Reference Maqueda, Willmott and Biggs2004; Tamura and others, Reference Tamura, Ohshima, Fraser and Williams2016). Polynyas are the main sources of such dense High Salinity Shelf Water (HSSW; Grumbine, Reference Grumbine1991). Sufficient negative buoyancy by salt rejection at the ocean surface triggers deep convection that conveys HSSW to the ocean floor, where it either flows towards the continental shelf slope or into the cavities below ice shelves. Beneath many ice shelves, HSSW may penetrate as far as the grounding line several hundred metres below sea level (Nicholls and others, Reference Nicholls, Østerhus, Makinson and Johnson2001). Since the convective and advective transfers are mainly adiabatic (without heat exchange), the potential temperature of HSSW remains close to the surface freezing point.
3.4 The three modes of ice-shelf melting
The freezing temperature of sea water decreases with increasing pressure and with increasing salinity (UNESCO, 1978; IOC and others, 2010). The HSSW temperature exceeds the in situ freezing point at depth by ~1°C at 1300 m (Jacobs and others, Reference Jacobs, Hellmer, Doake, Jenkins and Frolich1992) and constitutes a heat source available for latent heat of melting. In places where local turbulence or molecular diffusion transports sufficient heat and salt to the ice–water interface, the ice shelf melts. Submarine melting of ice shelves can be grouped into three categories, or ‘modes’ (see Fig. 1 in Jacobs and others, Reference Jacobs, Hellmer, Doake, Jenkins and Frolich1992). The HSSW driven mechanism is termed Mode 1 ice-shelf melting, which dominates the deep basal melting near the grounding line (Jacobs and others, Reference Jacobs, Hellmer, Doake, Jenkins and Frolich1992). Mode 2 ice-shelf melting is associated with the inflow of relatively warm Circumpolar Deep Water (CDW; Jacobs and others, Reference Jacobs, Hellmer, Doake, Jenkins and Frolich1992). Mode 3 ice-shelf melting is associated with Summer Surface Water near the ice-shelf front (Jacobs and others, Reference Jacobs, Hellmer, Doake, Jenkins and Frolich1992) which may be transported under the ice shelf by tidal pumping (e.g. Makinson and Nicholls, Reference Makinson and Nicholls1999; Joughin and Padman, Reference Joughin and Padman2003; Malyarenko and others, Reference Malyarenko, Robinson, Williams and Langhorne2019). Only Modes 1 and 3 ice-shelf melting are thought to lead to ISW formation and are thereby important for platelet-ice formation. This is because the CDW associated with Mode 2 melting is well above the surface freezing-point temperature (Jacobs and others, Reference Jacobs, Hellmer, Doake, Jenkins and Frolich1992).
3.5 ISW plumes and marine ice
As detailed above, basal melting can lead to ISW formation, characterised by cooling and freshening of the ambient water. As the water density at temperatures close to the freezing point mainly depends on salinity (UNESCO, 1981), freshening increases buoyancy, and the ISW plume rises along the basal slope of the ice shelf towards its front. The subsequent pressure release during the adiabatic ascent to shallower depths, together with the initial melt-induced freshening, raises the in situ freezing point of the plume. Therefore, when sufficient ascent and/or freshening occurs, the plume's freezing point can exceed its actual temperature, a condition termed in situ supercooling (Foldvik and Kvinge, Reference Foldvik and Kvinge1974, Reference Foldvik, Kvinge and Dunbar1977; Jacobs and others, Reference Jacobs, Fairbanks and Horibe1985).
Thermodynamic re-adjustment leads to the release of latent heat by the generation of individual ice crystals in the plume (Jenkins and Bombosch, Reference Jenkins and Bombosch1995; Smedsrud and Jenkins, Reference Smedsrud and Jenkins2004). However, the conditions under which nucleation occurs and frazil crystals are initially formed are still unclear. The growing ice crystals mixed with the sea water further increase the plume's buoyancy. This accelerates the ascent of water parcels along the ice slope, speeding up the pressure release (Jordan and others, Reference Jordan, Kimura, Holland, Jenkins and Piggott2015) and enhancing small-scale turbulent and diffusive processes. Theoretical considerations suggest a range of conceivable plume behaviours depending on the sensitive balance between crystal nucleation and precipitation, which itself is a function of growth rate (Rees Jones and Wells, Reference Rees Jones and Wells2018, Reference Rees Jones and Wells2015):
(1) Under conditions of near neutral crystal suspension and low growth rates, ascending water parcels sustain the increased buoyancy and may exit the cavity at shallower depths.
(2) Plumes under conditions where precipitation dominates experience an increase in bulk density. Arriving at the state of neutral buoyancy inevitably suppresses the generation of supercooling and associated frazil formation. Plumes could detach from the ice base and leave the cavity at depth, as observed near the front of the Ross Ice Shelf (Jacobs and others, Reference Jacobs, Gordon and Ardai1979, Reference Jacobs, Fairbanks and Horibe1985; Jacobs and Giulivi, Reference Jacobs, Giulivi, Spezie and Manzella1999).
(3) At faster growth rates, precipitation is more likely and crystals accumulate at the base of ice shelves (Rees Jones and Wells, Reference Rees Jones and Wells2018) as evident in observations (e.g. Fricker and others, Reference Fricker, Popov, Allison and Young2001; Craven and others, Reference Craven, Allison, Fricker and Warner2009; Kulessa and others, Reference Kulessa, Jansen, Luckman, King and Sammonds2014; Koch and others, Reference Koch, Fitzsimons, Samyn and Tison2015).
Such marine ice layers have been observed beneath several Antarctic ice shelves (e.g. Gow and Epstein, Reference Gow and Epstein1972; Morgan, Reference Morgan1972; Zotikov and others, Reference Zotikov, Zagardnov and Raikovsky1980; Oerter and others, Reference Oerter1992; Eicken and others, Reference Eicken, Oerter, Miller, Graf and Kipfstuhl1994; Tison and others, Reference Tison1998; Craven and others, Reference Craven, Allison, Fricker and Warner2009), and contribute to the stability of ice shelves by counteracting basal melting and closing basal crevasses, thus retarding calving events (Kulessa and others, Reference Kulessa, Jansen, Luckman, King and Sammonds2014). The overall process of ice-shelf melt and marine ice formation was termed ‘ice pump’ by Lewis and Perkin (Reference Lewis and Perkin1986), which in general terms describes a heat engine melting ice at some depth in the ocean and depositing it at a shallower location (Robin, Reference Robin1979; Lewis and Perkin, Reference Lewis and Perkin1983). Substantial evidence has been found that compacted marine ice is also the origin of occasionally observed green icebergs around Antarctica (Dieckmann and others, Reference Dieckmann, Hemleben and Spindler1987; Kipfstuhl and others, Reference Kipfstuhl, Dieckmann, Oerter, Hellmer and Graf1992, Fig. 2l), whose green colour is attributed to marine-derived organic matter included in the ice (Warren and others, Reference Warren1993), or, according to a more recent study, to iron-oxide minerals (Warren and others, Reference Warren, Roesler, Brandt and Curran2019).
3.6 Frazil crystals outside of the cavity
Generally, an ISW plume will continue to rise until it either loses its characteristic properties through mixing with other water masses, reaches a depth of neutral buoyancy or arrives at the ocean surface in front of an ice shelf. Clouds of frazil crystals suspended in supercooled ISW plumes at depth near ice-shelf cavities have been detected by acoustic methods (Penrose and others, Reference Penrose, Conde and Pauly1994) and retrieved by net hauls (Dieckmann and others, Reference Dieckmann, Rohardt, Hellmer and Kipfstuhl1986). The dependence of frazil concentration on supercooling is well known (Tsang and Hanley, Reference Tsang and Hanley1985), while the properties of frazil crystals and its occurrence are extensively reviewed in Daly (Reference Daly1984, Reference Daly1994). However, the only published studies we are aware of that successfully measured the size distribution, shape and concentration of frazil crystals were conducted in fresh water, primarily in rivers (e.g. Ghobrial and others, Reference Ghobrial, Loewen and Hicks2013; Marko and others, Reference Marko, Jasek and Topham2015; McFarlane and others, Reference McFarlane, Loewen and Hicks2017) or in the lab (e.g. Marko and Topham, Reference Marko and Topham2015).
3.7 Supercooling, platelet ice and sea ice
Frazil crystals that are advected out of ice-shelf cavities may eventually rise to the surface by their own individual buoyancy if they are not melted by overlying warmer water masses. Depending on where frazil crystals aggregate and grow, they interact differently with their surroundings, and have therefore been given distinct names. When accumulating underneath a solid sea-ice cover, crystals may form a semi-consolidated sub-ice platelet layer (Dayton and others, Reference Dayton, Robilliard and Devries1969; Gow and others, Reference Gow, Ackley, Weeks and Govoni1982; Crocker and Wadhams, Reference Crocker and Wadhams1989; Gough and others, Reference Gough2012b). If an in situ supercooled plume of ISW reaches the ocean surface (Hughes and others, Reference Hughes, Langhorne, Leonard and Stevens2014), crystals in such accumulations grow further (Leonard and others, Reference Leonard2006, Reference Leonard2011; Dempsey and others, Reference Dempsey2010; Smith and others, Reference Smith, Langhorne, Frew, Vennell and Haskell2012). Atmosphere driven heat extraction through the solid sea ice above freezes the water in the interstices between the individual crystals, leading to a downward progressing consolidation in the upper portions of the platelet layer, and ultimately to the platelets being incorporated into the growing sea-ice fabric (Dayton and others, Reference Dayton, Robilliard and Devries1969; Jeffries and others, Reference Jeffries, Weeks, Shaw and Morris1993; Gow and others, Reference Gow, Ackley, Govoni and Weeks1998; Smith and others, Reference Smith2001). This layer is called ‘incorporated platelet ice’ (Smith and others, Reference Smith2001) and has a distinct sea-ice crystal structure (e.g. Serikov, Reference Serikov1963; Eicken and Lange, Reference Eicken and Lange1989; Jeffries and others, Reference Jeffries, Weeks, Shaw and Morris1993; Tison and others, Reference Tison1998), which reflects the properties of the surface ocean during freezing (Gough and others, Reference Gough2012b; Langhorne and others, Reference Langhorne2015). The presence and persistence of a sub-ice platelet layer depends on the ratio between the flux of platelet crystals from below, and the growth rate of the overlying sea ice (Dempsey and others, Reference Dempsey2010; Mahoney and others, Reference Mahoney2011; Gough and others, Reference Gough2012b). Sea ice, mechanically strengthened by layers of incorporated platelet ice, can buttress and stabilise isolated ice tongues against neighbouring coastlines, and thus allow ice shelves to form outside of embayments (see Section 7; Hulbe and others, Reference Hulbe, Johnston, Joughin and Scambos2005; Massom and others, Reference Massom2010).
3.8 Anchor ice
Another sibling of platelet ice is anchor ice. It describes ice crystals covering the seafloor (Dayton and others, Reference Dayton, Robilliard and Devries1969; Mager and others, Reference Mager, Smith, Kempema, Thomson and Leonard2013). The continued presence of supercooled water allows these initial ice crystals to grow rapidly (Osterkamp and Gosink, Reference Osterkamp, Gosink, Barnes, Schell and Reimnitz1984) and form delicate, leaf-like ice structures that clump together (Kempema and others, Reference Kempema, Reimnitz, Clayton and Payne1993). This results in a patchy covering of ice that provides a lair for some benthic species, though it is also a potentially fatal environment for shallow water benthic communities (Dayton and others, Reference Dayton, Robilliard and Devries1969; Dayton, Reference Dayton1989; Denny and others, Reference Denny2011). These ice clusters can grow large enough to become buoyant and detach from the sea floor, lifting any adhered sediment, flora, fauna or scientific equipment into the overlying ice (Dayton and others, Reference Dayton, Robilliard and Devries1969; Hunt and others, Reference Hunt, Hoefling and Cheng2003). See Mager and others (Reference Mager, Smith, Kempema, Thomson and Leonard2013) for a review of anchor ice. Related to this effect and to platelet-ice formation, any ropes or instrumentation immersed into in situ supercooled water can also have ice form on them (Figs 2c and f), leading to measurement errors or instrument failures (Robinson and others, Reference Robinson, Grant, Stevens, Stewart and Williams2020).
4. Observational and numerical techniques
One of the main reasons why knowledge of platelet ice is so sparse is the challenge of obtaining in situ observations. The first obstacle to overcome is gaining access to coastal Antarctic sea ice, which is usually only possible from the few land-based stations close to the coast. In summertime, when icebreakers are able to get near the shoreline to supply Antarctic stations, the fast ice has usually either broken up, or the sub-ice platelet layers beneath it have already melted due to warm water inflow. Even after the coastal fast ice has been accessed, the presence of platelet ice is generally not immediately evident. Although there are a number of general approaches to determine regular sea-ice thickness (Haas and Druckenmiller, Reference Haas, Druckenmiller and Eicken2009), there are fewer direct and indirect techniques of obtaining in situ and remote observations of sub-ice platelet layers, and only one technique to determine the fraction of incorporated platelet ice. In the following, we divide these field techniques into ice-based, ocean-based and remote-sensing methods, based on where they are applied. We do not cover specific laboratory-based experimental investigations of platelet ice, as there have been very few of these due to the challenges of duplicating such a complex system in a laboratory (see e.g. Smith and others, Reference Smith, Gow and Langhorne2002). We also give a short overview of numerical techniques related to platelet-ice formation.
4.1 Ice-based measurements
Ice-based measurements have been used since the turn of the 20th century. They allow in situ observations and their relatively low operation costs (compared to techniques relying on aircraft or vessels) make them popular to this day. Most methods do however suffer from low spatio-temporal resolution, and all rely on ice conditions that are safe for on ice travel. Thus, observations of platelet ice during initial freeze-up or during spring time melt are rare and observations are often restricted to fast ice.
4.1.1 Drilling, thickness tapes and hot wires
The most direct and straightforward way to measure the thickness of sea ice in general, and the sub-ice platelet layer in particular, is by drilling holes into the sea ice. Sea ice can be drilled and cored with fairly simple and lightweight hand-powered equipment. A description of standard drilling equipment and field handling is found in Rand and Mellor (Reference Rand and Mellor1985) and Haas and Druckenmiller (Reference Haas, Druckenmiller and Eicken2009), while a detailed guide to thickness measurements using tapes and hot wires is given in Mahoney and Gearheard (Reference Mahoney and Gearheard2008). After a hole has been drilled through the ice, a thickness tape with a heavy metal bar (needed to penetrate the sub-ice platelet layer, if present) is lowered through the hole. Upon leaving the drillhole, the bar is pulled to the horizontal and catches at the bottom of the ice when the tape is pulled upwards (Mahoney and Gearheard, Reference Mahoney and Gearheard2008; Haas and Druckenmiller, Reference Haas, Druckenmiller and Eicken2009). To measure the thickness of the sub-ice platelet layer, the point where the first resistance to pulling is felt is noted as the lower boundary. The bar is then pulled upwards through the loose platelet crystals until it reaches the lower boundary of the consolidated ice, which is the upper boundary of the sub-ice platelet layer (Gough and others, Reference Gough2012b). Since the point at which resistance is first felt is somewhat subjective, this method can be ‘calibrated’ against camera footage if available, to get a better feeling for accuracy and precision (Gough and others, Reference Gough2012b). The measurement is complicated by the rather undefined platelet layer bottom, to the point where just individual crystals are suspended in the water. To measure sea-ice thickness over a longer period of time without the need to drill and re-drill access holes, the hot wire method can be used (Untersteiner, Reference Untersteiner1961; Mahoney and others, Reference Mahoney, Gearheard, Oshima and Qillaq2009). A weighted wire is lowered through an access hole and allowed to freeze in. A ground wire is allowed to freeze in a second hole close by. Both wires extend into the ocean. By applying an electrical current to the wires, they heat up and melt the surrounding ice. The weighted wire can then be pulled upwards until the weight catches at the underside of the ice. The amount of wire pulled up is measured, and the sea ice and sub-ice platelet layer thickness thus determined, before lowering the weight back to its original position. Problems with these methods include the weight getting stuck in the platelet layer when lowering the tape or wire back through the hole, and platelet ice forming on the suspended wire (Gough and others, Reference Gough2012b). The latter impacts the measured sub-ice platelet layer thickness, but not the measured thickness of the consolidated ice (Gough and others, Reference Gough2012b). Both thickness measurements with a thickness tape and with the hot wire method have the advantage of being inexpensive and easy to perform, allowing them to be used by non-experts (Mahoney and Gearheard, Reference Mahoney and Gearheard2008; Mahoney and others, Reference Mahoney, Gearheard, Oshima and Qillaq2009). However, both methods are time-consuming and not suitable for obtaining measurements at a high spatio-temporal resolution.
4.1.2 Thermistor chains
Thermistor chains are strings of temperature sensors which are lowered through an access hole in the ice and allowed to freeze in. They provide time series measurements of the temperature within the ice and of the water column beneath it, which can be used to calculate conductive heat fluxes and thermal conductivity (Trodahl and others, Reference Trodahl2000). The resulting energy budgets can be used to determine the amount of freezing due to heat loss to the atmosphere or to the ocean (Trodahl and others, Reference Trodahl2000; Smith and others, Reference Smith, Langhorne, Frew, Vennell and Haskell2012). Additionally, they can be used to infer the thickness evolution of the sea ice by examining when successive thermistors freeze in (Smith and others, Reference Smith, Langhorne, Frew, Vennell and Haskell2012; Hoppmann and others, Reference Hoppmann2015a). Different designs exist, with thermistors either housed in a metal tube filled with oil (Trodahl and others, Reference Trodahl2000; Smith and others, Reference Smith, Langhorne, Frew, Vennell and Haskell2012), mounted on a PVC rod (Perovich and Elder, Reference Perovich and Elder2001; Richter-Menge and others, Reference Richter-Menge2006) or mounted on a polycarbonate support (Pringle and others, Reference Pringle, Eicken, Trodahl and Backstrom2007; Gough and others, Reference Gough2012b). Newer designs feature digital temperature chips linked by flexible printed circuit boards, allowing probes up to 10 m length to be built (Zuo and others, Reference Zuo, Dou and Lei2018). A modified form of a thermistor chain was designed by Jackson and others (Reference Jackson2013), which is cheaper than standard ice mass-balance buoys (Richter-Menge and others, Reference Richter-Menge2006) since it does not depend on acoustic instruments to detect interfaces. Instead, resistors in the chain can be heated and the air, snow, consolidated ice, sub-ice platelet layer and ocean can be distinguished by differences in their thermal conductivity (Jackson and others, Reference Jackson2013). Hoppmann and others (Reference Hoppmann2015a) also used this feature to calculate the basal energy budget and infer the ice volume fraction in the sub-ice platelet layer.
The sea-ice thickness that can be monitored is limited by the length of the chain, which is typically between 2 and 5 m (Smith and others, Reference Smith, Langhorne, Frew, Vennell and Haskell2012; Hoppmann and others, Reference Hoppmann2015a; Zuo and others, Reference Zuo, Dou and Lei2018). Longer chains experience stronger shear strain from currents under the ice and are thus more prone to instrument failure. It is not clear if the ice forming in the drillhole surrounding the thermistor chain has different properties from the ambient ice and how this may affect temperature and heat conduction measurements (Trodahl and others, Reference Trodahl2000). Furthermore, the thermistor probe itself acts as a heat conductor (Trodahl and others, Reference Trodahl2000). The part of the hole which lies above sea level (the ice freeboard) usually does not refreeze, which needs to be taken into account. In addition, uncertainties in the measurements of platelet-layer thickness may result from ice forming on the chain. Thermistor chains offer a depth resolution dependent on the spacing of the thermistors and a quasi-continuous temporal resolution of sea-ice temperature and thickness. Due to the high temporal resolution, relatively low cost of operation and ease of deployment, thermistor chains have been a standard monitoring instrument for many years and continue to be so to this day, providing valuable long-term time series.
4.1.3 Coring and thin sectioning for incorporated platelet ice
The only reliable method currently available to measure the fraction of the incorporated platelet ice in the ice cover is to take a sea-ice core. The stratigraphy of that core can be analysed from thick and thin sections (Eicken and Lange, Reference Eicken and Lange1989; Jeffries and Weeks, Reference Jeffries and Weeks1992; Jeffries and others, Reference Jeffries, Weeks, Shaw and Morris1993; Tison and others, Reference Tison1998). The drilling action and subsequent removal of the core from the hole may dislodge an unknown amount of unconsolidated platelets from the bottom of the core, making any thickness measurement of the sub-ice platelet layer a minimum estimate. Thin sections of sea-ice cores can be routinely taken to investigate sea-ice crystal structure by determining c-axis orientation of the individual crystals (Fig. 5). The distribution of the c-axis orientation differs significantly between columnar and incorporated platelet ice. Standard procedures for both field and lab-based analysis of c-axis distribution are described in Langway (Reference Langway1959).
There are some automated methods for analysing thin sections using fabric analysers and dedicated software packages (e.g. Eicken, Reference Eicken1993; Wang and Azuma, Reference Wang and Azuma1999; Wilson and Sim, Reference Wilson and Sim2002; Wilson and Peternell, Reference Wilson and Peternell2011). Other methods of analysing crystal orientation in ice include X-ray diffraction (e.g. Owston, Reference Owston1958; Wenk, Reference Wenk, Kocks, Tomé, Wenk and Beaudoin2000; Miyamoto and others, Reference Miyamoto2005, Reference Miyamoto, Weikusat and Hondoh2011), with application to incorporated platelet ice in Obbard and others (Reference Obbard2016), and electron backscatter diffraction (EBSD; e.g. Iliescu and others, Reference Iliescu, Baker and Chang2004; Prior and others, Reference Prior2015; Wongpan and others, Reference Wongpan, Prior, Langhorne, Lilly and Smith2018c). The method suffers from the same spatio-temporal limitations as the other methods dependent on drilling, and further requires either a cold-lab close to the study site or temperature controlled shipment to a lab equipped with the necessary instruments, some of which are expensive.
4.1.4 Ground-based EM
Electromagnetic (EM) induction utilises the difference in electrical properties, particularly conductivity, between sea ice (Morey and others, Reference Morey, Kovacs and Cox1984) and ocean water to calculate sea-ice thickness using a single layer model (e.g. Haas and others, Reference Haas, Gerland, Eicken and Miller1997). The sub-ice platelet layer under sea ice has a mean volumetric salinity between that of sea ice and ocean water, acting as a second layer in the system. The return signal is difficult to interpret as the salinities, and thus the electromagnetic properties, of columnar ice and the sub-ice platelet layer do not differ as strongly as columnar ice from sea water.
Generally, single-frequency EM techniques either overestimate the thickness of the consolidated sea ice (Rack and others, Reference Rack, Haas and Langhorne2013) or underestimate the total sea-ice thickness (consolidated and sub-ice platelet layer, Hunkeler and others, Reference Hunkeler, Hendricks, Hoppmann, Paul and Gerdes2015). An approach using an inverse model and assuming three homogeneous layers (columnar ice, the sub-ice platelet layer and sea water) to interpret the response from a multi-frequency EM instrument is described in Hunkeler and others (Reference Hunkeler, Hendricks, Hoppmann, Paul and Gerdes2015, Reference Hunkeler, Hoppmann, Hendricks, Kalscheuer and Gerdes2016b). The resulting sub-ice platelet layer thickness depends on the ice volume fraction, the grain shape and the pore geometry in the layer, all of which are poorly constrained (Hunkeler and others, Reference Hunkeler, Hendricks, Hoppmann, Paul and Gerdes2015). A three layer model has also been applied in the case of a single-frequency device by optimising a forward model of the in-phase and quadrature components of the signal (Brett and others, Reference Brett2020). The thickness of the sub-ice platelet layer is the principal output, subject to the uncertainty in ice volume fraction. In theory, snow cover on the ice needs to be regarded as a fourth layer, though to our knowledge no studies exist using four layer models.
Ground-based EM induction systems can be stationary, for example in ice mass-balance stations, or towed. The former gives higher temporal resolution, the latter higher spatial resolution. Stationary EM stations can produce errors by disturbing the snow drift at the measurement site (personal communication from G. Leonard, 2019). All EM systems have a lower accuracy in shallow ocean environments (Haas, Reference Haas2006) and trade-offs must be made between resolution, penetration depth and signal-to-noise ratio (Haas, Reference Haas2006). It has to be kept in mind that EM systems do not produce point measurements but average over a footprint, the size of which depends on frequency, EM configuration and distance to the air–ice and ice–ocean interface (Reid and others, Reference Reid, Pfaffling and Vrbancich2006; Pfaffling and others, Reference Pfaffling, Haas and Reid2007). A great advantage of EM induction sounding is that it is a non-invasive method of measuring sea-ice thickness.
4.1.5 Oxygen isotopes (δ18O)
The isotopic composition of sea ice depends mainly on two factors: on the isotopic composition of the water from which it is formed, and on the growth rate of the ice (Souchez and others, Reference Souchez, Tison and Jouzel1987, Reference Souchez, Tison and Jouzel1988; Eicken, Reference Eicken and Jeffries1998). Sea ice is enriched in δ18O compared to the water it forms from and depleted in salt. This effect weakens with increasing sea-ice growth rate. No agreed protocol exists for the preparation of sea-ice samples for the analysis of stable isotopes oxygen-18 and deuterium (Smith and others, Reference Smith, Langhorne, Frew, Vennell and Haskell2012). To obtain a depth profile of isotope measurements, sea-ice cores are sliced horizontally, with each resulting sample stored in an airtight container and allowed to melt at room temperature. The resulting meltwater is poured into glass bottles and sealed for air-tightness. The liquid samples are then transported to a lab for isotope measurements. Full details of the procedure are given in Smith and others (Reference Smith, Langhorne, Frew, Vennell and Haskell2012). Samples can be analysed with dual-inlet, continuous flow or cavity ring-down laser mass spectrometers, but these systems differ in precision to each other with the dual-inlet giving a higher precision than the continuous flow mass spectrometers (Toyota and others, Reference Toyota2013). Isotope measurements on platelet crystals from the sub-ice platelet layer pose particular issues because of the likelihood that a thin film of sea water freezing on each platelet-ice crystal will alter the results of the value attributed to the single crystal. Although attempts have been made to examine the differences between methods for preparing bulk and single platelet crystals for isotope analysis (personal communication from Natalie Robinson, by email, 2010), to our knowledge nothing has been published on these methods so far.
The relationship between freezing rate, δ18O and bulk salinity (Tison and others, Reference Tison1998) was used to model growth rates as a function of sea-ice δ18O values relative to the δ18O value of the sea water from which it formed (Eicken, Reference Eicken and Jeffries1998). Several modifications of this model exist, attempting to account for glacial meltwater in ISW, the high growth rate of platelet ice compared to columnar ice (Smith and others, Reference Smith, Langhorne, Frew, Vennell and Haskell2012) and the possible regime shift in fractionation between low and high growth rates (Toyota and others, Reference Toyota2013).
4.2 Ocean-based measurements
4.2.1 Water sampling
The space between the individual crystals within the porous sub-ice platelet layer is filled with sea water, usually referred to as pore water or interstitial water. Although a myriad of methods exist to obtain samples for the biogeochemical studies of sea ice (Miller and others, Reference Miller2015), obtaining water samples from the interstices between the platelet crystals is not easily possible, especially not without significantly disturbing the system. A potential solution was presented by Dieckmann and others (Reference Dieckmann, Arrigo and Sullivan1992), who developed a promising device for high-resolution under-ice sampling that can be lowered through a core hole in the ice to sample the unconsolidated platelet layer. It is essentially an array of small tubes guided through a plastic pipe that can be inserted into the platelet layer to simultaneously obtain samples of interstitial water at different depths. It was successfully utilised by Arrigo and others (Reference Arrigo1995) and Robinson and others (Reference Robinson, Arrigo, Kolber, Gosselin and Sullivan1998) to study the biogeochemistry and photophysiology of the platelet-ice community (Miller and others, Reference Miller2015). Despite its simple and low-cost design, this technique has however not been utilised widely.
4.2.2 Acoustics
Acoustic Doppler Current Profilers (ADCPs) are a standard oceanographic instrument to measure ocean velocities. They also record backscatter intensity, which is related to the size and concentration of scatterers in the water column, though it is difficult to distinguish between the two (and impossible for single-frequency instruments; Gartner, Reference Gartner2004). The measured backscatter intensity is related to particle concentration and size by using empirically derived regression models (e.g. Ghobrial and others, Reference Ghobrial, Loewen and Hicks2012) or theoretical backscatter models. These depend on particle size, shape and material properties and the accuracy of the sonar, all of which may not be well constrained (Ghobrial and others, Reference Ghobrial, Loewen and Hicks2013). The same principles and caveats apply to ice-profiling sonars (IPSs), which can be used to monitor sea-ice draft and frazil ice in the water column (Ito and others, Reference Ito2015). As far as we are aware, Ito and others (Reference Ito2015), who observed supercooling and increased backscatter in an Arctic polynya, remains the only study to have linked IPS backscatter to frazil ice presence in the ocean. The same principle, linking backscatter occurring simultaneously with supercooling and platelet-ice formation to the presence of frazil in the water column, was used by Leonard and others (Reference Leonard2006) to interpret ADCP data. However, both with IPSs and ADCPs, care must be taken not to interpret vertical migration of zooplankton or tidal advection of particulate matter as changes in the supercooling and frazil ice regime (Leonard and others, Reference Leonard2006). Furthermore, difficulties arise due to backscatter from particles other than frazil ice (Marko and others, Reference Marko, Jasek and Topham2015) and ice build-up on the instruments (e.g. Leonard and others, Reference Leonard2006).
There have been efforts to measure particle concentration and size distribution of suspended frazil in the laboratory (Ghobrial and others, Reference Ghobrial, Loewen and Hicks2012; Marko and Topham, Reference Marko and Topham2015) and in rivers (e.g. Morse and Richard, Reference Morse and Richard2009; Ghobrial and others, Reference Ghobrial, Loewen and Hicks2013; Marko and others, Reference Marko, Jasek and Topham2015) using single-, two- and multi-frequency backscatter, respectively, but to our knowledge no such measurements have been published for sea water. The exception is the study of Purdie (Reference Purdie2012), in which the authors were able to calculate estimates of particle mass and concentration from single-frequency backscatter, but not size.
Although the spatial resolution of IPS and ADCP measurements is low, they can offer very high temporal resolution. The length of the record depends on the deployment technique. Instruments moored to the sea ice or deployed through an access hole (e.g. Leonard and others, Reference Leonard2006; Mahoney and others, Reference Mahoney2011; Stevens and others, Reference Stevens2014) can only be deployed and recovered from sea ice that is sufficiently stable, whereas floating buoys and instruments moored on the sea bed (e.g. Ito and others, Reference Ito2015) can be deployed from a vessel, during low or absent sea-ice cover, thereby giving information during freeze-up and melt. Bottom-mounted ADCPs and IPSs can monitor the water column below and the underside of drifting ice (Eulerian view), whereas instruments moored to the sea ice will move with the ice (Lagrangian view). IPSs and ADCPs are both expensive and the analysis of the data complicated. At present, the most reliable ice information from both instruments is total sea-ice draft (including the sub-ice platelet layer) and the presence of frazil in the water column. A recent approach to map sea-ice draft and roughness at high resolutions used a phase measuring bathymetric sonar mounted on an autonomous underwater vehicle (AUV) (1 m and 5 cm respectively; Lucieer and others, Reference Lucieer, Nau, Forrest and Hawes2016). The sea-ice roughness was successfully related to sub-ice platelet layer presence. This method is able to retrieve information on the underside of the sea ice over large areas, though the deployment of AUVs is costly (see Section 4.2.4 on more information on AUVs) and the processing of bathymetric sonar data is complicated.
4.2.3 Cameras
Independent of the particular method that is used to detect or measure the properties of sub-ice platelet layers, it is always useful to also co-deploy underwater cameras to gain a visual impression of the subject in focus (see e.g. Fig. 2a). From simple waterproof action cameras to pipe- or sewage cameras to complex, high-tech camera systems, any camera can give additional value to other measurements. Camera systems can be used to position instruments relative to the bottom of the sub-ice platelet layer (Smith and others, Reference Smith2001). Leonard and others (Reference Leonard2006) and Smith and others (Reference Smith, Langhorne, Frew, Vennell and Haskell2012) used still images from a video camera, positioned such that it viewed a platelet crystal at the bottom of the sub-ice platelet layer in front of a ruler, to measure growth rates of that crystal. Cameras can also be used to estimate frazil crystal size (Gough and others, Reference Gough2012b), and their temporal occurrence (Hoppmann and others, Reference Hoppmann2015b). By using cross-polarised filters, frazil crystal size distributions and the fraction of disk shaped crystals can be measured (McFarlane and others, Reference McFarlane, Loewen and Hicks2017), though no such measurements exist for marine settings.
As far as we are aware, no dedicated studies exist which employ cameras mounted on marine mammals to map sub-ice platelet layer presence, although the sub-ice platelet layer can occasionally be seen in video footage from such mammals (Davis and others, Reference Davis1999; Figs 2j and k). Studies employing cameras mounted on AUVs or remotely operated vehicles (ROVs) would be more useful, and are described below.
Ice algae concentrations can be studied using camera systems operating in the visible or hyperspectral range (Cimoli and others, Reference Cimoli, Meiners, Lund-Hansen and Lucieer2017) as well as by measuring the irradiance under sea ice (Fritsen and others, Reference Fritsen, Wirthlin, Momberg, Lewis and Ackley2011; Wongpan and others, Reference Wongpan2018b). Positioning instruments such as cameras in supercooled water incurs the risk of ice build-up on the instrument (as seen in Figs 2c and f) and as with all drillhole-based measurements, spatio-temporal resolution is low. Additionally, cameras cannot measure sub-ice platelet layer thickness, unless they are combined with some sort of measurement stick or ruler (see Fig. 2a). The advantage of cameras is that they can examine the sub-ice platelet layer, and especially individual crystals, virtually undisturbed. In late 2017, the McMurdo Oceanographic Observatory (MOO) was installed 21 m below the sea ice of McMurdo Sound, consisting of a live-streaming HD video camera that can point and zoom in all directions. Including cameras operated by divers, this project has created a wealth of underwater footage and currently represents (to our knowledge) the most extensive collection of openly accessible material (Cziko, Reference Cziko2019).
4.2.4 Robotic vehicles
Robotic vehicles such as ROVs and AUVs have a higher spatial and temporal range than sampling for sub-ice platelets by drilling or using divers. However, none of these vehicles can detect incorporated platelet-ice or sub-ice platelet layer thickness, but platelet layer presence and floating frazil crystals may be detected using upward looking camera systems (Spears and others, Reference Spears2016) or sonars (Lucieer and others, Reference Lucieer, Nau, Forrest and Hawes2016). On-board acoustic sensors, such as ADCPs, could be used to detect frazil in the water column. Furthermore, supercooling can be investigated using an on-board CTD system (Nelson and others, Reference Nelson2017), though not all currently integrated CTD systems have a high enough resolution to do this reliably.
There exists a wide variety of robotic vehicles that have been successfully deployed in ice-covered environments (see e.g. Spears and others, Reference Spears2016, for an overview of some of these systems). Gliders are AUVs that vary their buoyancy to produce forward propulsion and can travel under sea ice (Asper and others, Reference Asper2011). However, without access to the surface, the glider position can only be estimated. This means that, although gliders can detect potential or in situ supercooling in the water column under sea ice or ice shelves, this information cannot be linked to a precise enough location (Nelson and others, Reference Nelson2017). Different methods to improve under-ice navigation exist (Leonard and Bahr, Reference Leonard, Bahr, Dhanak and Xiros2016) and are included in some systems (Webster and others, Reference Webster, Lee and Gobat2014), but the only deployments to date beneath ice shelves using navigation are beneath the Dotson Ice Shelf where there was little-to-no in situ supercooling observed (personal communication from Pierre Dutrieux by email, 2019). ROVs rely on a tether for communication and power supply and are thus limited in range compared to AUVs.
A problem common to all vehicles is the high risk of loss should ice form on or in the instrument itself, as well as a large number of other causes (e.g. Strutt, Reference Strutt2006). Vehicles relying on buoyancy for forward or vertical propulsion may become trapped by strong currents or vertical stratification (Asper and others, Reference Asper2011; Nelson and others, Reference Nelson2017). A number of ROVs exist that can be deployed through drillholes in the ice, such as Icefin (Spears and others, Reference Spears2016; Meister and others, Reference Meister2018), SCINI (Cazenave and others, Reference Cazenave, Zook, Carroll, Flagg and Kim2011), Deep-SCINI (Burnett and others, Reference Burnett, Rack, Zook and Schmidt2015) and a customised Ocean Modules M500 ROV (Katlein and others, Reference Katlein, Arndt, Belter, Castellani and Nicolaus2019). Larger AUVs and ROVs require significant logistical effort for deployment and recovery, often ship based, especially when relying on moored acoustic sources for navigation, making under ice missions rare and expensive.
4.2.5 SCUBA divers
Due to its presence mainly at the underside of coastal Antarctic sea ice, the only means to perform in situ controlled and targeted sampling of sub-ice platelet layers is by SCUBA-diving missions based from Antarctic stations. This is especially true for biological sampling. However, because of the challenging logistics and the dangers for human life that come with diving in ice-covered seas, there are only a few studies so far that have collected data using SCUBA divers. These have been performed on a regular basis in the shallow areas of McMurdo Sound, but were mostly focused on the benthic realm. The earliest work involving extensive diving in supercooled waters is the one of Dayton and others (Reference Dayton, Robilliard and Devries1969), who showed that anchor ice frozen to the seabed is of ‘considerable biological significance’ because it can lift benthic organisms from the seafloor into the sub-ice platelet layer. They also observed instantaneous ice crystal formation in the water while diving. Later studies include for example the ones of Dayton (Reference Dayton1989) and Denny and others (Reference Denny2011) at McMurdo and Galea and others (Reference Galea2016) in Atka Bay (Fig. 2d). More recently, the MOO has been installed and was continuously maintained by divers (Cziko, Reference Cziko2019). Many of the health risks associated with diving under Antarctic ice can be mitigated by using ROVs, however this is equally challenging from a logistical perspective.
4.3 Airborne remote sensing
Airborne remote sensing forms the link between in situ observations and satellite remote sensing and is crucial for verifying satellite mission data. Currently only one method of airborne observations of platelet ice exists, which is EM by plane or helicopter (Rack and others, Reference Rack, Haas and Langhorne2013). Airborne EM functions in the same way as ground-based EM systems described above, but has a much higher spatial coverage and higher operating costs. A sub-ice platelet layer has been observed in EM thickness estimates below the sea ice in front of the McMurdo Ice Shelf and is in evidence beneath the ice shelf to a thickness of 55 m: the maximum ice-shelf thickness detectable by the airborne EM (induction sounding) instrument (Rack and others, Reference Rack, Haas and Langhorne2013). The larger footprint of the measurement means that small-scale features cannot be resolved. In addition to the much wider spatial coverage, an advantage over ground-based EM is that airborne EM does not require a minimum sea-ice thickness to operate safely, allowing measurements during freeze in and melt/break-up. This method has great potential to fill the current observational gaps especially in remote regions far from coastal research stations, but much more development work with respect to suitable inversion algorithms is necessary.
4.4 Satellite remote sensing
Satellite altimetry measures the elevation of the snow or ice upper boundary above mean sea level. This measurement may then be converted to sea-ice thickness using estimates of snow depth and density, and sea-ice density (e.g. Kurtz and Markus, Reference Kurtz and Markus2012). Global Navigation Satellite System (GNSS) surface elevation data have been used to assess the influence that the presence of a sub-ice platelet layer has on estimates of sea-ice thickness, with overestimates in consolidated sea-ice thickness of up to 19% close to an ice-shelf edge (Price and others, Reference Price2014). Indeed there is strong evidence of the influence on ICESat laser altimeter data of a sub-ice platelet layer beneath multi-year sea ice in McMurdo Sound between 2003 and 2009 (Price and others, Reference Price, Rack, Haas, Langhorne and Marsh2013). If developed and used in conjunction with other tools, satellite altimetry has the potential to provide an Antarctic-wide view of the pervasiveness of platelet ice.
4.5 Frazil ice numerical models
Frazil ice formation in the water column influences the buoyancy of the surrounding water parcel (e.g. Jordan and others, Reference Jordan, Kimura, Holland, Jenkins and Piggott2015) and thus its dynamics as well as potentially influencing sea ice by accumulating as platelet ice. However, the processes involved in frazil ice formation are not well understood and its transport in supercooled plumes is difficult to observe. In addition, the range of scales on which these processes occur, from the molecular scale nucleation to ISW plumes extending hundreds of kilometres, naturally excludes them from being explicitly simulated in the same model for computational reasons. Frazil ice and plume models aim to bridge this gap by providing much needed insights into large scale effects of these small-scale physical processes. Their results can be used to parameterise frazil ice processes in large scale numerical models such as coupled ice-shelf–ocean, Earth system or climate models. Early theoretical work on frazil ice dynamics was undertaken by Daly (Reference Daly1984). There are many numerical models dealing with the representation of frazil generated by supercooled water resulting from heat loss to the atmosphere, e.g. in polynyas, but this section will focus on models dealing with frazil precipitating from ISW. Models focus on the propagation of (frazil-laden) ISW plumes and the effects on marine-ice thickness and sea-ice thickness (e.g. Jenkins and Bombosch, Reference Jenkins and Bombosch1995; Smedsrud and Jenkins, Reference Smedsrud and Jenkins2004; Holland and Feltham, Reference Holland and Feltham2005) and have been steadily improved since then (e.g. Hughes and others, Reference Hughes, Langhorne, Leonard and Stevens2014; Jordan and others, Reference Jordan, Kimura, Holland, Jenkins and Piggott2015; Cheng and others, Reference Cheng2019). The newest frazil ice models more closely investigate the formation of the individual crystals (e.g. Rees Jones and Wells, Reference Rees Jones and Wells2018) based on theoretical investigations of nucleation (Rees Jones and Wells, Reference Rees Jones and Wells2015). Unfortunately, the lack of quantitative observations of frazil ice properties (e.g. frazil crystal size distributions and concentrations, and basal drag parameterisations) and the knowledge gaps in crucial physical processes such as nucleation mean that verification of model findings is difficult to impossible.
4.6 Platelet-ice numerical models
Since platelet ice is difficult and costly to measure on large scales, as seen above, many groups have worked on numerical models describing platelet ice (e.g. Ohashi and others, Reference Ohashi, Sasaki and Yoshimura2004; Ohashi and Kawano, Reference Ohashi and Kawano2007; Dempsey, Reference Dempsey2008; Kawano and Ohashi, Reference Kawano and Ohashi2008; Dempsey and others, Reference Dempsey2010; Wongpan and others, Reference Wongpan, Langhorne, Dempsey, Hahn-Woernle and Sun2015; Buffo and others, Reference Buffo, Schmidt and Huber2018). Since the exclusion of platelet-ice effects from sea-ice models leads to large errors in areas affected by platelet-ice accretion (Buffo and others, Reference Buffo, Schmidt and Huber2018), these efforts are not only of relevance to the platelet-ice community, but also to the wider Antarctic sea ice and climate modelling community. Currently, the most comprehensive 3-D model of platelet-ice accretion is the one by Wongpan and others (Reference Wongpan, Langhorne, Dempsey, Hahn-Woernle and Sun2015), which is however limited to modelling small 10 cm3 volumes at the ice–water interface, due to high computational costs. The most comprehensive 1-D model, capable of re-creating ice core characteristics (columnar to incorporated platelet-ice transition, thickness and growth duration) by forcing with realistic ocean and atmosphere parameters, was created by Buffo and others (Reference Buffo, Schmidt and Huber2018). In the presence of platelet ice, the authors intend the model to calculate sea-ice properties accurately from environmental parameters. However, to date, this has only been tested against the single set of observations used to configure the model.
4.7 Ocean–ice shelf numerical models
In addition to the challenges in modelling ISW plumes, frazil formation and platelet-crystal accretion described above, accurately simulating the precursor processes of platelet-ice formation and incorporation is difficult. On the one hand, these processes affect the large scale circulation via the generation and advection of ISW and supercooled ocean water. On the other hand, they depend on molecule- to centimetre scale processes, such as ice-shelf melting, ocean turbulence and double diffusion. The evolution of the ocean boundary layer beneath the ice shelf–ocean interface is not well captured in current ocean and ice-shelf models. Sub-ice-shelf ocean observations are critically needed to constrain this component in model solutions and improve the underlying algorithms.
In the past decade, many numerical ocean model frameworks have adopted combined algorithmic and parameterised representations of thermodynamic ice–ocean interaction (e.g. MITgcm, Losch, Reference Losch2008; FESOM, Timmermann and others, Reference Timmermann, Wang and Hellmer2012; Fluidity, Kimura and others, Reference Kimura, Candy, Holland, Piggott and Jenkins2013; ROMS, Robertson, Reference Robertson2013; NEMO, Mathiot and others, Reference Mathiot, Jenkins, Harris and Madec2017; MOM6, Adcroft and others, Reference Adcroft2019). However, a detailed digest of the many caveats in modelling ice-shelf cavities is beyond the scope of this study. A comprehensive overview on the evolution in theoretical concepts and numerical technologies of ice-shelf ocean simulation, including the main contemporary challenges, is given in Williams and others (Reference Williams, Jenkins, Determann, Jacobs and Weiss1998) and Dinniman and others (Reference Dinniman2016). To date, there have been very few measurements of salinity, temperature, currents or anything else beneath ice shelves with which ocean–ice shelf numerical models can be tested.
5. Geographical occurrence and seasonality
We will now give an overview of the published observations of platelet ice in the Southern Ocean, building on the map produced by Langhorne and others (Reference Langhorne2015). An updated and expanded version of this map is shown in Figure 4. Again we neglect the Arctic, since our definition of platelet ice applies to the Antarctic only. We will discuss possible causes for the presence or absence of platelet ice in different regions and link this to larger scale processes around Antarctica.
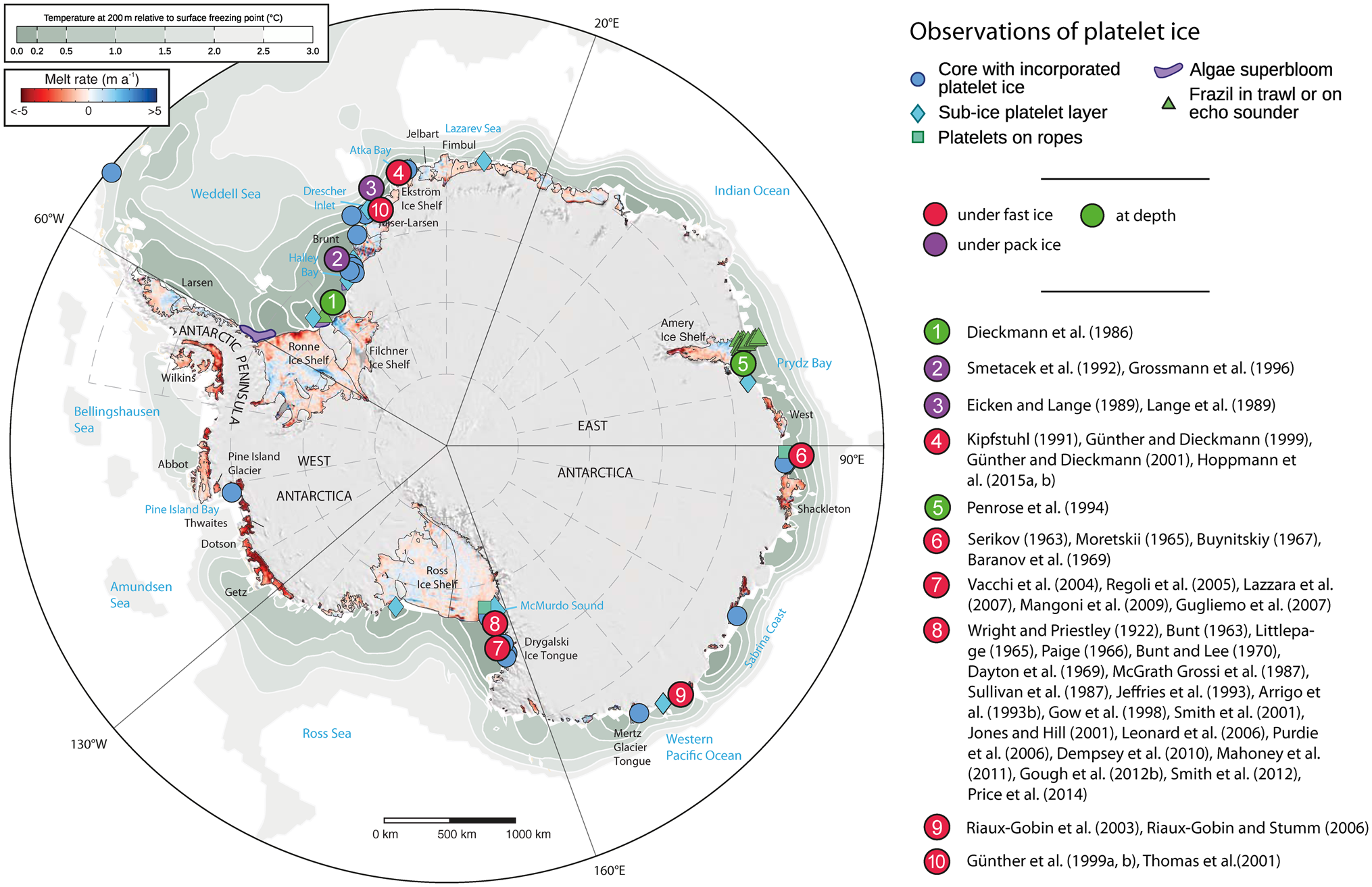
Fig. 4. Observations of platelet ice around Antarctica following Langhorne and others (Reference Langhorne2015), and background of potential ocean temperature at 200 m relative to surface freezing point. Ice shelves and their basal mass budgets, colour coded from <-5 m a−1 (melting) to > +5 m a−1 (freezing), and 2009 Moderate Resolution Imaging Spectroradiometer (MODIS) mosaic of Antarctica from Rignot and others (Reference Rignot, Jacobs, Mouginot and Scheuchl2013).
5.1 Weddell Sea and Filchner–Ronne Ice Shelf
Sub-ice platelet layers and associated incorporated platelet ice in sea-ice cores have mainly been observed close to the ice-shelf edge or coastal polynyas (Eicken and Lange, Reference Eicken and Lange1989; Lange and others, Reference Lange, Ackley, Wadhams, Dieckmann and Eicken1989; Smetacek and others, Reference Smetacek1992). However, Dai and others (Reference Dai2010) found platelet ice in drifting pack ice at the northwestern rim of the Weddell Sea Gyre, far from the influence of ice shelves. The Weddell Sea Gyre (Deacon, Reference Deacon1979; Fahrbach and others, Reference Fahrbach, Rohardt, Schröder and Strass1994) may have carried the ice floe from its point of formation to the position it was observed. In front of the Filchner–Ronne Ice Shelf, ice crystals with diameters between 10 and 25 mm have been captured by trawl nets at depths of 250 m (Dieckmann and others, Reference Dieckmann, Rohardt, Hellmer and Kipfstuhl1986).
5.2 Southeastern Weddell Sea
In Atka Bay close to the Ekström Ice Shelf, incorporated platelet ice has been observed in all fast-ice cores that have been published so far (e.g. Kipfstuhl, Reference Kipfstuhl1991; Günther and Dieckmann, Reference Günther and Dieckmann1999, Reference Günther and Dieckmann2001; Hoppmann and others, Reference Hoppmann2015a, Reference Hoppmann2015b; Arndt and others, Reference Arndt, Hoppmann, Schmithüsen, Fraser and Nicolaus2020). The contribution of platelet ice to sea-ice thickness is sometimes so high that cores may not contain any columnar ice (Hoppmann and others, Reference Hoppmann2015a, Reference Hoppmann2015b; Arndt and others, Reference Arndt, Hoppmann, Schmithüsen, Fraser and Nicolaus2020), although this mainly depends on the timing of initial sea-ice formation. At the end of a typical sea-ice growth season, the sub-ice platelet layer is on average 4 m thick, with up to 10 m observed locally (Hoppmann and others, Reference Hoppmann2015a, 2015b; Hunkeler and others, Reference Hunkeler, Hoppmann, Hendricks, Kalscheuer and Gerdes2016b; Arndt and others, Reference Arndt, Hoppmann, Schmithüsen, Fraser and Nicolaus2020). Hoppmann and others (Reference Hoppmann2015b) found evidence of episodic bursts of floating frazil crystals in the water column that are most likely tidal-induced, and resulting from a plume of supercooled ISW emerging from the Ekström Ice Shelf cavity (Smith and others, Reference Smith2020). Comparable sub-ice platelet layer thicknesses (3–7 m) were observed 10–15 km from the coast in Drescher Inlet (e.g. Eicken and Lange, Reference Eicken and Lange1989; Günther and others, Reference Günther, Gleitz and Dieckmann1999b). This layer was found to thin offshore and eastwards to ~0.5 m 35 km offshore of Halley Bay. Incorporated platelet ice was found to make up 11% of the total sea-ice thickness in the area with some cores consisting of 50% incorporated platelet ice (Eicken and Lange, Reference Eicken and Lange1989). The sub-ice platelet layer was observed using cameras mounted on an ROV (Marschall, Reference Marschall1988) which suggested that the platelets were mainly advected from deeper in the water column but also grew in situ (Eicken and Lange, Reference Eicken and Lange1989).
5.3 East Antarctica
At the Amery Ice Shelf, Penrose and others (Reference Penrose, Conde and Pauly1994) recovered frazil crystals from depths of 150 m using trawl nets. Along the East Antarctic coast, platelet ice is intensively formed at the Antarctic shore off Mirny Station and is responsible for the anomalously rapid growth of the fast ice there and its friable structure (Serikov, Reference Serikov1963; Moretskii, Reference Moretskii1965). These authors also concluded that Antarctic platelet ice is formed by supercooling of sea water by ice shelves and icebergs. To our knowledge, there are no other studies that found individual icebergs to be a significant source for platelet-ice formation, although this may locally be the case under favourable conditions similar to those described in Section 3. The platelet ice off Mirny station attaches to the underside of the fast ice there and, after break-up, is carried by currents out to a distance of more than 40 km from the shore (Moretskii, Reference Moretskii1965). The great thickness of the fast ice at Mertz Glacier has been hypothesised to be caused, at least in part, by platelet-ice accretion, though no direct evidence for this exists (Massom and others, Reference Massom2010). Platelet ice has been found close to Dumont D'Urville Station (Delille and others, Reference Delille, Jourdain, Borges, Tison and Delille2007), which may have been formed from ISW advected from Mertz Glacier (Williams and Bindoff, Reference Williams and Bindoff2003).
5.4 Ross Sea and McMurdo Sound
Observations of platelet ice in McMurdo Sound, eastern Ross Sea, were compiled by Langhorne and others (Reference Langhorne2015). The first ever observations of platelet ice were made in McMurdo Sound (Hodgson, Reference Hodgson1907) and it remains the region with the longest time series and the largest number of observations, mainly under fast ice (Langhorne and others, Reference Langhorne2015). Observations include: sub-ice platelet layers (e.g. Bunt, Reference Bunt1963; Gow and others, Reference Gow, Ackley, Weeks and Govoni1982; SooHoo and others, Reference SooHoo1987; Crocker, Reference Crocker1988; Rivkin and others, Reference Rivkin, Putt, Alexander, Meritt and Gaudet1989; Smith and others, Reference Smith2001; Ryan and others, Reference Ryan2006; Hughes and others, Reference Hughes, Langhorne, Leonard and Stevens2014; Price and others, Reference Price2014; Brett and others, Reference Brett2020); incorporated platelet ice (e.g. Gow and others, Reference Gow, Ackley, Govoni and Weeks1998; Smith and others, Reference Smith, Langhorne, Trodahl, Haskell and Cole1999, Reference Smith2001; Jones and Hill, Reference Jones and Hill2001; Dempsey and others, Reference Dempsey2010; Smith and others, Reference Smith, Langhorne, Frew, Vennell and Haskell2012; de Jong and others, Reference de Jong2013); the presence of frazil crystals in the water column (e.g. Leonard and others, Reference Leonard2006; Gough and others, Reference Gough2010, Reference Gough2012b); anchor ice (e.g. Dayton and others, Reference Dayton, Robilliard and Devries1969; Mager and others, Reference Mager, Smith, Kempema, Thomson and Leonard2013, and references therein) and ice on ropes (e.g. Hodgson, Reference Hodgson1907; Wright and Priestley, Reference Wright and Priestley1922; Dayton and others, Reference Dayton, Robilliard and Devries1969; Barry, Reference Barry1988; Leonard and others, Reference Leonard2006; Gough and others, Reference Gough2010; Mahoney and others, Reference Mahoney2011). Due to the local ocean circulation (e.g. Leonard and others, Reference Leonard2006; Mahoney and others, Reference Mahoney2011; Robinson and others, Reference Robinson, Williams, Stevens, Langhorne and Haskell2014), in the western McMurdo Sound platelet ice occurs earlier in the season, and makes up a greater percentage of the sea-ice thickness compared to the eastern McMurdo Sound, where it is sometimes absent (Dempsey and others, Reference Dempsey2010; Hughes and others, Reference Hughes, Langhorne, Leonard and Stevens2014). In areas with strong tidal currents the sub-ice platelet layer has been observed to be separated from the overlying sea ice, and moving independently of the stationary fast ice (Robinson and others, Reference Robinson, Stevens and McPhee2017). The long time series of platelet-ice observations in McMurdo Sound (over 100 years) does not show any trends in the surface ocean temperatures or the thickness of the platelet ice (Langhorne and others, Reference Langhorne2015).
5.5 West Antarctica
No platelet ice has been observed in the Amundsen and Bellingshausen Seas, except for the observation of platelet ice in Pine Island Bay in the winter of 1991/92 by Veazey and others (Reference Veazey, Jeffries and Morris1994). That was the first study of sea ice in the region and the temperature profiles from the area, the first taken in 1994 (Jacobs and others, Reference Jacobs, Hellmer and Jenkins1996, Reference Jacobs, Jenkins, Giulivi and Dutrieux2011), suggest that Veazey and others (Reference Veazey, Jeffries and Morris1994) may have observed one of the last occurrences of in situ supercooled water outflow from Pine Island Glacier. This hypothesis remains speculative however, since the beginning of the oceanographic and glaciological observational record at Pine Island Bay possibly coincides with the beginning, or at least acceleration, of the dramatic melt-induced thinning (Wingham and others, Reference Wingham, Wallis and Shepherd2009) of the glacier due to ocean forcing and associated changes in cavity geometry (Jenkins and others, Reference Jenkins2010; Jacobs and others, Reference Jacobs, Jenkins, Giulivi and Dutrieux2011). The warming and shoaling of CDW in the Amundsen and Bellingshausen Seas (Schmidtko and others, Reference Schmidtko, Heywood, Thompson and Aoki2014), which in 1994 was already present at the Pine Island Glacier calving front and was significantly warmer than the freezing point (Jenkins and others, Reference Jenkins, Vaughan, Jacobs, Hellmer and Keys1997), make the presence of ISW and platelet ice highly unlikely in present-day conditions.
5.6 Discussion of observations
To understand the distribution of platelet-ice occurrence described above, we will take a closer look at the precursor of platelet-ice formation, the formation of ISW through ice-shelf melting. The presence of ISW is a necessary, though not sufficient precursor for platelet-ice formation (Mahoney and others, Reference Mahoney2011). The occurrence of supercooling strongly depends on the mode of melting (see Section 3.4). In the Ross and Weddell Seas, the shelf waters are cold (e.g. Nicholls and others, Reference Nicholls, Osterhus, Makinson, Gammelsrod and Fahrbach2009; Orsi and Wiederwohl, Reference Orsi and Wiederwohl2009) and platelet ice has been observed there (Langhorne and others, Reference Langhorne2015). In contrast, for the Amundsen and Bellingshausen Seas (e.g. Jacobs and others, Reference Jacobs, Hellmer and Jenkins1996, Reference Jacobs, Jenkins, Giulivi and Dutrieux2011) and along the Sabrina Coast (Silvano and others, Reference Silvano, Rintoul, Peña-Molino and Williams2017), the continental shelf is flooded by warm CDW and there are no recent observations of platelet ice (Langhorne and others, Reference Langhorne2015). Even in close proximity to ice-shelf cavities cold enough to produce ISW, platelet ice may be absent for all or part of the year. This can be due to (a) ISW emerging at depth; (b) ISW mixing with warmer surface water masses before exiting the cavity (Jacobs and others, Reference Jacobs, Hellmer, Doake, Jenkins and Frolich1992; Hattermann and others, Reference Hattermann, Nøst, Lilly and Smedsrud2012); (c) in situ supercooling in the ISW plume being relieved through latent heat release from marine or anchor ice formation at depth (Sections 3.5 and 3.8) or (d) the presence of a polynya with intense brine rejection, as hypothesised by Langhorne and others (Reference Langhorne2015). The absence of platelet-ice observations in front of parts of the Filchner–Ronne (Nicholls and others, Reference Nicholls, Osterhus, Makinson, Gammelsrod and Fahrbach2009; Hattermann and others, Reference Hattermann, Nøst, Lilly and Smedsrud2012), the Amery (Shi and others, Reference Shi, Cheng, Jiao and Hou2010; Zheng and others, Reference Zheng, Shi, Jiao and Ge2011) and the Ross Ice Shelves (Jacobs and others, Reference Jacobs, Fairbanks and Horibe1985; Jeffries and Adolphs, Reference Jeffries and Adolphs1997; Nelson and others, Reference Nelson2017) is likely attributed to (a). In cases (a), (b) and (c), ISW may be in situ supercooled at some depth and frazil ice can form. However, stratification may prevent the frazil from rising to the surface and reaching the sea ice by causing the crystals to melt in the overlying warmer waters. For example, ISW has been observed at depth in front of the Ross Ice Shelf (e.g. Jacobs and others, Reference Jacobs, Fairbanks and Horibe1985; Nelson and others, Reference Nelson2017), but Jeffries and Adolphs (Reference Jeffries and Adolphs1997) did not observe any platelet ice there. In the hypothesised case (d), frazil crystals suspended at depth in the in situ supercooled ISW plume may be melted by the heat source of pressurised HSSW once deep convection sets in. Furthermore, crystals that have risen to the ocean surface may be indistinguishable from the frazil or granular ice formed during intense atmospheric cooling and polynya sea-ice production, as suggested by Langhorne and others (Reference Langhorne2015).
5.7 Seasonality of formation
The seasonality of platelet-ice formation is likely to be different for each location and may indeed vary from year to year depending on factors such as icebergs influencing fast-ice persistence and ocean circulation (Brunt and others, Reference Brunt, Sergienko and MacAyeal2006; Dinniman and others, Reference Dinniman, Klinck and Smith2007; Remy and others, Reference Remy, Becquevort, Haskell and Tison2008; Robinson and others, Reference Robinson, Williams, Barrett and Pyne2010; Mahoney and others, Reference Mahoney2011). Platelet-ice formation from July to September was recorded in several locations in the Mirny area (Buynitskiy, Reference Buynitskiy1967), and has also been seasonally observed in other regions (Wright and Priestley, Reference Wright and Priestley1922; Dayton and others, Reference Dayton, Robilliard and Devries1969; Crocker and Wadhams, Reference Crocker and Wadhams1989; Günther and Dieckmann, Reference Günther and Dieckmann1999), suggesting that the water only becomes supercooled during this period or after sea ice has begun to form. However, there are substantial deviations from these dates in other regions. The mode of melting below the Amery Ice Shelf is known to be seasonal, with Mode 1 dominating melting and Mode 2 contributing in winter (Herraiz-Borreguero and others, Reference Herraiz-Borreguero2015). ISW has been found at mid-depth year round and marine ice formation is highest during winter (Herraiz-Borreguero and others, Reference Herraiz-Borreguero, Allison, Craven, Nicholls and Rosenberg2013). In Atka Bay, Hoppmann and others (Reference Hoppmann2015a, Reference Hoppmann2015b) found a thickening sub-ice platelet-ice layer throughout winter with a decline in thickness starting in December with the arrival of warm surface waters. In McMurdo Sound, the onset of near surface supercooling conditions was observed as early as March, with platelet ice appearing as early as April (Leonard and others, Reference Leonard2006) in a year when the local oceanography was heavily influenced by a grounded iceberg in the Ross Sea (Brunt and others, Reference Brunt, Sergienko and MacAyeal2006; Dinniman and others, Reference Dinniman, Klinck and Smith2007; Remy and others, Reference Remy, Becquevort, Haskell and Tison2008; Robinson and others, Reference Robinson, Williams, Barrett and Pyne2010; Mahoney and others, Reference Mahoney2011; Robinson and Williams, Reference Robinson and Williams2012). Other researchers found ISW arrived as late as mid-July and platelet ice began to form in early August (Mahoney and others, Reference Mahoney2011; Gough and others, Reference Gough2012b). The onset of supercooling conditions in McMurdo Sound is influenced by the location in the Sound, with an outflow of ISW likely present throughout the sea-ice growth season in western McMurdo Sound, as evident in oceanographic observations (e.g. Robinson and others, Reference Robinson, Williams, Stevens, Langhorne and Haskell2014) and the high contribution of platelet ice in sea-ice cores (e.g. Barry, Reference Barry1988; Dempsey and others, Reference Dempsey2010, recorded an absence of columnar ice from sea-ice cores taken in western McMurdo Sound). In the eastern McMurdo Sound, a summertime inflow of warmer surface waters prevents the appearance of surface supercooling at the start of the sea-ice growth season (Barry, Reference Barry1988; Barry and Dayton, Reference Barry and Dayton1988; Robinson and others, Reference Robinson, Williams, Stevens, Langhorne and Haskell2014). This warm inflow subsides during winter as the ISW plume exiting in western McMurdo Sound spreads eastwards (Barry and others, Reference Barry, Dayton, Dunbar and Leventer-Reed1990; Leonard and others, Reference Leonard2006; Mahoney and others, Reference Mahoney2011; Robinson and others, Reference Robinson, Williams, Stevens, Langhorne and Haskell2014) resulting in an onset of platelet-ice occurrence deeper in the core (later in the growth season) for cores taken successively further eastwards (e.g. Hughes and others, Reference Hughes, Langhorne, Leonard and Stevens2014).
The picture of platelet-ice presence painted above should be viewed with some caution. Both the geographical and temporal occurrence suffer from significant sampling bias, with a higher density of observations close to research stations and during conditions with well-established fast ice. In addition to this, the picture is most likely incomplete, with many incidental observations of platelet ice never published. To expand the base of observations beyond platelet-ice observations published in the literature, it would be essential to undertake a search of expedition reports and other such sources. However, this was far beyond the scope of this review.
6. Physical properties
Incorporated platelet ice differs from columnar sea ice in the orientation of the individual ice crystals making up the sea-ice fabric. In columnar ice, the crystals are aligned, whereas in incorporated platelet ice, the crystals are randomly oriented (Fig. 5). Since the sub-ice platelet layer is a loose accretion of solid ice platelets surrounded and permeated by sea water, the physical property in which the sub-ice platelet layer differs most from consolidated sea ice (columnar and incorporated platelet ice) is the ice-volume fraction, also sometimes termed solid volume fraction. Most other differences in properties result from the difference in ice-volume fraction. In the following, we will examine some of the physical properties of platelet ice more closely.
6.1 Size of individual platelets
Platelet crystals attached to a slab of sea ice removed from McMurdo Sound in 1999 were reported by Smith and others (Reference Smith2001) to be ~2 mm thick and up to 200 mm in diameter. Systematic measurements of the size distribution of individual platelet crystals were published by Stevens and others (Reference Stevens, O'Connor and Robinson2019), where over 100 individual platelet crystals were measured. The crystals were predominantly within the 50–100 mm diameter class, with some up to ~200 mm in diameter. Thicknesses ranged from ~0.5 to 4 mm.
6.2 Ice-volume fraction
The correct interpretation of signals from radar and EM induction measurements of sea ice are dependent on a knowledge of the electrical properties of sea ice. They are discussed in Morey and others (Reference Morey, Kovacs and Cox1984) and a field approach using a multi-frequency EM instrument is described in Hunkeler and others (Reference Hunkeler, Hendricks, Hoppmann, Paul and Gerdes2015, Reference Hunkeler, Hoppmann, Hendricks, Kalscheuer and Gerdes2016b). The electrical conductivity of the sub-ice platelet layer can be used to calculate its porosity ϕ, and subsequently its ice-volume fraction β = 1 – ϕ, via a modified Archie's law (Archie, Reference Archie1942; Hunkeler and others, Reference Hunkeler, Hoppmann, Hendricks, Kalscheuer and Gerdes2016b) given by $$\phi = \left({\displaystyle{{ \sigma_{\rm p} } / {\sigma_{\rm b}} }} \right)^{1/m}$$ The ice-volume fraction β thereby depends on a knowledge of the brine conductivity, σ b, the conductivity of the sub-ice platelet layer, σ p and an empirical cementation factor, m. The latter in turn depends on the pore geometry and connectivity, and on the shape of the platelet crystals. All these factors are not well constrained for the sub-ice platelet layer (Hunkeler and others, Reference Hunkeler, Hoppmann, Hendricks, Kalscheuer and Gerdes2016b). A range of other techniques have also been used to try to measure or estimate the ice-volume fraction in the sub-ice platelet layer. Thus it is not surprising that estimates of the ice-volume fraction, collected in Table 2, range from 0.16 (Price and others, Reference Price2014) to >0.50 (Jeffries and others, Reference Jeffries, Weeks, Shaw and Morris1993), where the former used Archimedes' Law and the latter examined horizontal thin sections of incorporated platelet ice and determined what proportion of the ice was platelet crystals when frozen in.
Table 2. Estimates for the ice-volume fraction β within the sub-ice platelet layer

Table modified from Gough and others (Reference Gough2012b) and Hoppmann and others (Reference Hoppmann2015b).
6.3 Platelet crystal growth rates
Through video camera still analysis, Smith and others (Reference Smith, Langhorne, Frew, Vennell and Haskell2012) reported that individual crystals in the sub-ice platelet layer grew in episodic bursts with growth rates of the order of 10−6 m s−1 during these bursts. Incorporated platelet ice was reported by Smith and others (Reference Smith, Langhorne, Frew, Vennell and Haskell2012) to have growth rates of the order of 10−7 m s−1, an order of magnitude slower than the individual platelet crystals. Leonard and others (Reference Leonard2006) reported maximum growth rates for individual platelet crystals in the sub-ice platelet layer that were close to the minimum crystal growth rate observed by Smith and others (Reference Smith, Langhorne, Frew, Vennell and Haskell2012).
6.4 Basal topography and ocean dynamics in the boundary layer
The presence of sub-ice platelet layers forms an enhanced basal topography and increases the drag at the underside of sea ice (McPhee and others, Reference McPhee, Stevens, Smith and Robinson2016; Robinson and others, Reference Robinson, Stevens and McPhee2017) to 6–30 times the value for sea ice unaffected by platelet ice (Robinson and others, Reference Robinson, Stevens and McPhee2017). The growth of platelet ice is hypothesised to itself be limited by turbulent heat transfer, a quantity dependent on drag. Robinson and others (Reference Robinson, Stevens and McPhee2017) described the effect of platelet-ice roughness-induced mixing on limiting (in a stratified ocean with warmer water underlying a supercooled plume) or increasing (in a homogeneous ocean or thick supercooled plume) platelet-ice growth. This also applies to vertical convection due to the latent heat and salt fluxes associated with platelet-ice growth (Robinson and others, Reference Robinson, Williams, Stevens, Langhorne and Haskell2014). From heat flux calculations, Smith and others (Reference Smith2001) inferred a kinematic eddy viscosity for a sub-ice platelet layer to be ε = 2 × 10−5 m2 s−1. Stevens and others (Reference Stevens, Robinson, Williams and Haskell2009) measured average turbulent energy dissipation rates beneath McMurdo Sound fast ice, where platelet ice is commonly present, to be 3 × 10−8 m2 s−3.
6.5 Crystal c-axis distribution
c-axis distribution measured from thin sections of ice cores reveal differences between columnar ice and incorporated platelet ice (Weeks and Ackley, Reference Weeks and Ackley1982; Gough and others, Reference Gough2012b; Fig. 5). In columnar ice, c-axes are preferentially oriented in the horizontal, forming a girdle structure when plotting their orientation (e.g. Weeks and Ackley, Reference Weeks and Ackley1982; Gough and others, Reference Gough2010, Reference Gough2012b). In cases where the columnar ice grows in the presence of a current of constant direction, the horizontal c-axes cluster in the direction of the current (Langhorne and Robinson, Reference Langhorne and Robinson1986; Gough and others, Reference Gough2010). Incorporated platelet ice shows random c-axis orientations, with some even aligned in the vertical (e.g. Gough and others, Reference Gough2012b). The orientation of platelet crystals is thought to be dependent on the rotation of the crystal as it settles at the underside of the ice (Dempsey and others, Reference Dempsey2010; Wongpan and others, Reference Wongpan, Langhorne, Dempsey, Hahn-Woernle and Sun2015, Reference Wongpan, Prior, Langhorne, Lilly and Smith2018c). Wongpan and others (Reference Wongpan, Prior, Langhorne, Lilly and Smith2018c) were able to measure the crystallographic a-axis orientations and showed that these too show differences between columnar ice and platelet ice.

Fig. 5. Selected thin sections along a sea-ice core from McMurdo Sound, Antarctica, viewed under cross-polarised light, showing granular, columnar and incorporated platelet ice. Depth from sea-ice surface in metres. Crystallographic c-axes orientations have been displayed for three horizontal sections at 0.60, 1.38 and 1.56 m. The blue dots correspond to the orientation of the c-axes when projected on to an upper hemisphere Schmidt net, a standard method of visualising c-axes orientation (Langway, Reference Langway1959). Horizontal c-axes will plot along the perimeter of the circle whereas vertical c-axes will lie in the centre. Vertical thin sections are shown for 0.06–1.15, 1.20–1.29, 1.84–1.93 and 2.20–2.29 m.
6.6 Salinity, permeability and brine volume
Through analysis of multiple sea-ice cores taken at one site, Gough and others (Reference Gough, Mahoney, Langhorne, Williams and Haskell2012a) showed that the salinity of incorporated platelet ice is slightly higher than the salinity of columnar ice, after growth rates were taken into account. This is a subtle effect that is not clearly obvious in the C-shaped salinity profiles from single cores (Smith and others, Reference Smith, Langhorne, Frew, Vennell and Haskell2012). Wongpan and others (Reference Wongpan, Hughes, Langhorne and Smith2018a) showed that this is the result of the arithmetic mean permeability for columnar and incorporated platelet ice being indistinguishable beneath thick Antarctic landfast sea ice, implying that porosity is more important than crystal geometry. It should be noted that Wongpan and others (Reference Wongpan, Hughes, Langhorne and Smith2018a) were only able to probe incorporated platelet ice which has a porosity similar to columnar sea ice. Less is known of the sub-ice platelet layer which has been measured to be isothermal. If it is assumed that the system is in thermal equilibrium, then the salinity gradient in the brine in the interstitial space of the sub-ice platelet layer must also be minimal. Diffusive transport is unlikely to dominate in such a system. At the time of writing, the permeability-porosity relationship and fluid transport in the sub-ice platelet layer are poorly understood.
6.7 Mechanical properties
Many authors (e.g. Weeks, Reference Weeks2010) have shown that all mechanical properties of sea ice are strongly dependent on the porosity, and platelet ice is no exception. Thus the mechanical properties of incorporated platelet ice would be expected to be very similar to the properties of columnar sea ice. Measurements of flexural strength and the fatigue properties of the first-year landfast sea ice of McMurdo Sound were made throughout the 1990s by subjecting in situ cantilever beams to repeated bending with zero mean stress (e.g. Haskell and others, Reference Haskell, Robinson and Langhorne1996; Langhorne and others, Reference Langhorne, Squire, Fox and Haskell1998; Langhorne and Haskell, Reference Langhorne and Haskell1999). These beams were comprised mainly of columnar ice, but included between 10 and 35% incorporated platelet ice (Langhorne and others, Reference Langhorne2015). The crystallographic structure was shown to exert much less influence on the mechanical properties of the beams than salinity, temperature or brine volume (e.g. Langhorne and Haskell, Reference Langhorne and Haskell2004). In contrast, in marine ice with low bulk salinities, the anisotropy in crystal fabric controls the rheological behaviour when samples are subject to unconfined uniaxial compression (Dierckx and others, Reference Dierckx, Peternell, Schroeder and Tison2014). Indeed, recently formed marine ice appears to behave as natural isotropic ice, provided the higher temperature of marine ice is taken into account (Dierckx and Tison, Reference Dierckx and Tison2013). To our knowledge, no data on mechanical properties of the high porosity sub-ice platelet layer have been published.
6.8 Oxygen isotopes (δ18O)
There are two main controls on the δ18O values of sea ice: (i) the growth rate of the sea ice (higher growth rates lead to lower sea-ice δ18O values); and (ii) the δ18O values of the water which the ice formed from (lower parent water values lead to lower sea-ice δ18O values). As noted above, individual platelet crystals grow in episodic bursts at rates that are an order of magnitude faster than columnar ice. It would therefore be expected that δ18O values for crystals from the sub-ice platelet layer and for incorporated platelet ice would be lower than for columnar ice; however, this is not the case. Smith and others (Reference Smith2015) reported that there was no discernible difference between the isotopic composition of columnar ice and incorporated platelet ice. Reliable δ18O measurements for platelet crystals from the sub-ice platelet layer have not been published. Smith and others (Reference Smith2001) reported measurements from such crystals, but noted the possible contamination from the presence of sea water. Attempts to minimise this contamination have been made but not yet published (personal communication from Natalie Robinson). Smith and others (Reference Smith2015) reported a range of derived effective fractionation coefficients (the ratio of the isotopic composition of the sea water to that of the sea ice) to be +1.84‰ to +2.21‰ for McMurdo Sound sea ice in 2009.
7. Significance and impact
7.1 Contribution to sea-ice thickness and volume
Sea-ice thickness in front of an ice shelf is influenced by the stabilisation of the upper water column by glacial meltwater. Using an ocean model with ice-shelf cavity thermodynamics, Hellmer (Reference Hellmer2004) predicted that sea ice is up to 0.2 m thicker for this reason. The overall thickening effect of glacial meltwater emerging from the ice-shelf cavity on sea ice is likely to be higher than 0.2 m since the model of Hellmer (Reference Hellmer2004) does not take into account supercooling, the formation of frazil ice, or platelet ice. Since approximately half of Antarctica's coastline is fringed by ice shelves (Fretwell and others, Reference Fretwell2013), this potentially affects large areas of the Southern Ocean. In the Ross Sea, Jeffries and others (Reference Jeffries, Weeks, Shaw and Morris1993) determined that incorporated platelet ice constituted over half of the thickness of fast ice in some locations in McMurdo Sound. In late winter, in first-year sea ice close to the ice shelf, at least 0.25 m of the 2 m thick cover forms due to heat loss to the ocean (Purdie and others, Reference Purdie, Langhorne, Leonard and Haskell2006; Gough and others, Reference Gough2012b). Similarly, multi-year fast ice attached to the Mertz Glacier tongue was estimated to be between 10 and 55 m thick, and frazil accumulation must have contributed to its thickness (Massom and others, Reference Massom2010).
The percentage contribution of platelets to the total volume of sea ice has been estimated for various regions in Antarctica. Kipfstuhl (Reference Kipfstuhl1991) determined that fast ice in Atka Bay contained up to 50% incorporated platelet ice, while Günther and Dieckmann (Reference Günther and Dieckmann1999) found up to 60% of the fast ice to consist of incorporated platelet ice in the same area. Jeffries and others (Reference Jeffries, Weeks, Shaw and Morris1993) and Smith and others (Reference Smith2001) showed that platelet ice can add up to more than 40% of the total sea-ice mass in McMurdo Sound. Eicken and Lange (Reference Eicken and Lange1989) found their cores from the coastal zones of the southeastern Weddell Sea to contain 11% of incorporated platelet ice, while Lange (Reference Lange1988) and Lange and others (Reference Lange, Ackley, Wadhams, Dieckmann and Eicken1989) estimate that between 20 and 30% of sea ice in coastal regions is comprised of platelet ice. The spatial variability of the contribution of platelet ice to sea ice was studied by Barry (Reference Barry1988), Smith and others (Reference Smith2001) and Dempsey and others (Reference Dempsey2010) based on ice-core analyses. The thickness of sub-ice platelet layers, when present, have been reported to range from consisting of ranging from individual crystals attached to the ice bottom (Mahoney and others, Reference Mahoney2011) to 8 m or thicker (Hughes and others, Reference Hughes, Langhorne, Leonard and Stevens2014; Price and others, Reference Price2014; Hoppmann and others, Reference Hoppmann2015a). In some locations on the eastern side of McMurdo Sound, incorporated platelet ice has been observed in the lower parts of cores only (e.g. Smith and others, Reference Smith2001; the lower 690 mm in a core with a total length of 2150 mm), with some locations not having any incorporated platelet ice present at certain times (e.g. Cape Evans in Smith and others, Reference Smith2001). In contrast, Hoppmann and others (Reference Hoppmann2015a) and Hughes and others (Reference Hughes, Langhorne, Leonard and Stevens2014) took sea-ice cores in Atka Bay and western McMurdo Sound, respectively, which consisted of incorporated platelet ice throughout, i.e. these cores did not contain any pure columnar ice.
7.2 Further effects on sea ice
Sea ice is a critical part of Earth's climate system through its high albedo (especially when snow covered), thermal insulation properties, and through its role in deep water formation. It is generally agreed that the growth of a sub-ice platelet layer causes sea ice to be thicker than it would be with heat loss to the atmosphere only (e.g. Smith and others, Reference Smith, Langhorne, Frew, Vennell and Haskell2012; Hoppmann and others, Reference Hoppmann2015b, Section 7.1). The presence of platelet ice will therefore alter the thermal insulation properties of sea ice and its longevity, with flow-on effects to climate. The overall impact of ice-shelf basal melt on sea-ice formation, and hence on climate, is not well understood. The contribution of ice-shelf melting as a factor in the unexplained discrepancy between global climate models and satellite-derived sea-ice extent around Antarctica has been explored using different climate models, but is controversial (Bintanja and others, Reference Bintanja, van Oldenborgh, Drijfhout, Wouters and Katsman2013, Reference Bintanja, van Oldenborgh and Katsman2015; Swart and Fyfe, Reference Swart and Fyfe2013; Holland and others, Reference Holland2014; Pauling and others, Reference Pauling, Bitz, Smith and Langhorne2016, Reference Pauling, Smith, Langhorne and Bitz2017; Bronselaer and others, Reference Bronselaer2018). As explained in Section 4.7, no ice-shelf–ocean (and thus climate) models currently include the effect of platelet-ice formation on sea-ice extent and thickness, so these papers have only taken into account the direct effects of the freshwater input (e.g. stratification, and sometimes also cooling). As shown earlier in this paper, manifestations of ice shelf–ocean interaction and associated phenomena have been observed in many locations around Antarctica (Fig. 4). Although aspects of these interactions have been investigated in detail for example in McMurdo Sound (Ross Sea) and Atka Bay (Weddell Sea), little is known about the overall role of platelet ice for coastal sea ice in Antarctica. This lack of regional information is a barrier for investigating the effects of platelet ice on sea ice and the climate system.
Although it is relatively simple to accurately predict the growth of undeformed Arctic sea ice by thermodynamic modelling, a thermodynamic model which accurately predicts ice growth in the Arctic will not do the same in the Antarctic when platelet ice is present due to the negative ocean heat flux associated with the presence of platelet ice (Langhorne and others, Reference Langhorne2015). This phenomenon has encumbered efforts to predict growth rates of fast ice in Antarctica ever since Crocker and Wadhams (Reference Crocker and Wadhams1989), as detailed in Smith and others (Reference Smith2015).
7.3 Effects on ice-shelf buttressing
As noted in previous sections, incorporated platelet ice results in thicker sea ice and therefore must contribute considerably to the overall stability of the fast ice around Antarctica and to its prolonged existence, especially in bays and inlets. The stability of the fast ice, with its estimated area of between 5.5 × 105 and 8 × 105 km2 (Kozlovsky and others, Reference Kozlovsky, Nazintsev, Fedotov and Cherepanov1977, as cited in Fedotov and others, Reference Fedotov, Cherepanov, Tyshko and Jeffries1998) also plays an important role in the stability of ice shelves, for example by protecting the calving front from ocean swell (Massom and others, Reference Massom2018) or through buttressing (MacAyeal and Holdsworth, Reference MacAyeal and Holdsworth1986). Consequently, the melting of ice shelves resulting in platelet-ice formation forming thicker and more stable fast ice may indirectly lead to ice shelves that are more stable due to protective, more stable fast ice in front of them. However, further research is needed to investigate these connections. The climate effects of platelet ice are therefore complex, involving a series of feedbacks.
The stability of fast ice and ice shelves not only has consequences for the global climate system, but also for the ecology of the coastal regions, as explained in Section 7.5.
7.4 An indicator for ice-shelf processes
More recently, Hoppmann and others (Reference Hoppmann2015b) and in much more detail, Langhorne and others (Reference Langhorne2015), hypothesised that the presence and evolution of platelet ice and its physical properties as a signature of supercooling could be an indicator for the state of an ice shelf with respect to processes in the ice-shelf cavity. Smith and others (Reference Smith2015) suggested using δ18O samples from sea ice as evidence for ice-shelf melt processes, but noted the challenges of doing this. This is a particular useful and intriguing idea, especially since the sampling beneath Antarctic ice shelves is a huge logistical and financial challenge. Although the exact connection between those two cryospheric elements is not clear, it is certain that the properties of sea ice (or more accurately, fast ice) close to an ice shelf should be an important element in the monitoring of the latter.
7.5 Significance for ecosystems and ecology
The first studies of the biology associated with sub-ice platelet layers date back to the 1960s in McMurdo Sound (Bunt, Reference Bunt1963; Bunt and Wood, Reference Bunt and Wood1963; Bunt and Lee, Reference Bunt and Lee1970). These first explorations described the dense microbial assemblages associated with platelet layers (e.g. Palmisano and Sullivan, Reference Palmisano, SooHoo and Sullivan1985; Dieckmann and others, Reference Dieckmann, Arrigo and Sullivan1992; Arrigo and others, Reference Arrigo, Robinson and Sullivan1993b; Ackley and Sullivan, Reference Ackley and Sullivan1994; Arrigo and Thomas, Reference Arrigo and Thomas2004). The high concentration of biological matter in sub-ice platelet layers is usually immediately evident by the reddish-brown colour of the interstitial waters, and has significant implications for the entire coastal Antarctic food web.
7.5.1 Phytoplankton and bacteria
The colonisation of the sub-ice platelet layer by microalgae and other small planktonic organisms probably commences concurrently with its formation (Günther and Dieckmann, Reference Günther and Dieckmann1999). Whether the platelet crystals themselves are instrumental in scavenging the organisms from the water column is still not clear, albeit very likely (Garrison and Sullivan, Reference Garrison, Ackley and Buck1983, Reference Garrison, Close and Reimnitz1988; Dieckmann and others, Reference Dieckmann, Rohardt, Hellmer and Kipfstuhl1986; Walker and Marchant, Reference Walker and Marchant1989). Other evidence of particle inclusions is known from marine ice (Kipfstuhl and others, Reference Kipfstuhl, Dieckmann, Oerter, Hellmer and Graf1992; Goodwin, Reference Goodwin1993; Warren and others, Reference Warren1993; Eicken and others, Reference Eicken, Oerter, Miller, Graf and Kipfstuhl1994; Craven and others, Reference Craven, Allison, Fricker and Warner2009), where resuspended sediment particles may be incorporated in a similar manner. The organisms either dwell while suspended within the interstitial water between the platelets, or grow attached to platelet crystals themselves (Smetacek and others, Reference Smetacek1992). Late summer sub-ice platelet layers seem to constitute a protected refugium for ice-associated algae and bacteria during a phase of intense pack-ice melting.
The timing of a phytoplankton bloom is strongly affected by light conditions, primarily controlled by the properties of the overlying snow cover (McGrath Grossi and others, Reference McGrath Grossi, Kottmeier, Moe, Taylor and Sullivan1987; Palmisano and others, Reference Palmisano, SooHoo and Sullivan1985; Wongpan and others, Reference Wongpan2018b). The spectral irradiance regime is generally the main limitation for growth of bottom-ice and under-ice microalgae (Palmisano and Sullivan, Reference Palmisano, SooHoo and Sullivan1985; Palmisano and others, Reference Palmisano, SooHoo and Sullivan1985, Reference Palmisano, Beeler SooHoo and Sullivan1987; Cota and Smith, Reference Cota and Smith1991), and light levels in the sub-ice platelet layer are lower than in the congelation ice above (Bunt, Reference Bunt2013b). The role of light in controlling algal growth and production in sub-ice platelet layers has been studied intensively, and there is evidence of extreme shade adaptation in sub-ice platelet layer associated algae (Palmisano and Sullivan, Reference Palmisano, SooHoo and Sullivan1985; Palmisano and others, Reference Palmisano, Beeler SooHoo and Sullivan1987; Arrigo and others, Reference Arrigo, Dieckmann, Gosselin and Sullivan1991, Reference Arrigo, Robinson and Sullivan1993b; Robinson and others, Reference Robinson, Arrigo, Iturriaga and Sullivan1995; Lizotte and Sullivan, Reference Lizotte and Sullivan1991; Guglielmo and others, Reference Guglielmo2000; Lazzara and others, Reference Lazzara, Nardello, Ermanni, Mangoni and Saggiomo2007; Mangoni and others, Reference Mangoni2008). However, other factors seem to compensate the light limitation, strongly favouring algal growth in this habitat: stable temperatures near the freezing point of sea water, stable salinity, a continuous supply of nutrients, ample opportunity for colonisation as well as protection from large grazers such as krill (Thomas and Dieckmann, Reference Thomas and Dieckmann2002a).
Algal communities particularly thrive in layers that are hydraulically connected to the water column, supplying nutrients and providing seed populations of microalgae (Arrigo, Reference Arrigo and Thomas2016; Meiners and others, Reference Meiners2018; van Leeuwe and others, Reference van Leeuwe2018). Being the most porous of all sea-ice habitats, sub-ice platelet layers provide a large surface area and at the same time enable free nutrient exchange with the underlying sea water (Arrigo and Thomas, Reference Arrigo and Thomas2004; Bunt, Reference Bunt2013a).
The productivity and biomass accumulation in sub-ice platelet layers often exceeds the high concentrations measured in the lower few centimetres of congelation ice (Bunt and Lee, Reference Bunt and Lee1970; Kottmeier and others, Reference Kottmeier, Grossi and Sullivan1987; McGrath Grossi and others, Reference McGrath Grossi, Kottmeier, Moe, Taylor and Sullivan1987; Smetacek and others, Reference Smetacek1992; Arrigo and others, Reference Arrigo, Kremer and Sullivan1993a; Arrigo and others, Reference Arrigo, Robinson and Sullivan1993b, Reference Arrigo1995; Günther and Dieckmann, Reference Günther and Dieckmann1999; Lazzara and others, Reference Lazzara, Nardello, Ermanni, Mangoni and Saggiomo2007; Meiners and others, Reference Meiners2012). Seasonal peaks in chlorophyll-a (Chl-a) concentrations in Antarctic and Arctic sea-ice types are up to 5400 and 800 mg Chl-a m−3, respectively (Meiners and others, Reference Meiners2012; Arrigo, Reference Arrigo and Thomas2016). Reported peak accumulation in sub-ice platelet layer habitats are >6000 mg Chl-a m−3, higher than any other sea-ice habitat either in the Arctic or the Antarctic (Arrigo and others, Reference Arrigo, Mock, Lizotte, Thomas and Dieckmann2010). In a recent comprehensive synopsis of Chl-a standing stocks in Antarctic landfast ice, Meiners and others (Reference Meiners2018) described how sites with platelet ice have the greatest concentration of sea-ice algal biomass in these highly productive regions.
Grossmann and others (Reference Grossmann, Lochte and Scharek1996) showed that substantial heterotrophic potential can be established within this special habitat. Nutrient profiles and small-scale distribution of algal and bacterial cells suggest a close coupling between these two groups of organisms, thus implying the establishment of a community structure. In areas of greatest biomass accumulation, the platelet layers are also good examples of where the ‘chemostatic’ ice algal production can take place (Thomas and others, Reference Thomas, Lizotte and Arrigo1998): the high biomass production in highly porous systems and in the absence of grazers is supported by rapid turnover of inorganic nutrients. This is fuelled by high concentrations of organic matter which results in high-bacterial nitrogen, phosphorus and silicate regeneration in an elevated pH medium (Thomas and Dieckmann, Reference Thomas and Dieckmann2002b; Ichinomiya and others, Reference Ichinomiya2008).
A consequence of these sites of intensive biological activity is that there is considerable flux of material out of the platelet layers to the underlying waters and sediments. This has been measured by the deployment of small sediment traps under platelet layers and much of the material falling out is made up of ice diatoms, sometimes contained within faecal pellets produced by protists and small metazoans (Günther and others, Reference Günther, George and Gleitz1999a, Reference Günther, Gleitz and Dieckmann1999b; Riaux-Gobin and others, Reference Riaux-Gobin, Tréguer, Poulin and Vétion2000, Reference Riaux-Gobin2005; Thomas and others, Reference Thomas2001; Ichinomiya and others, Reference Ichinomiya2008).
7.5.2 Grazers in platelet ice
Thomas and Dieckmann (Reference Thomas and Dieckmann2002b) describe the debate about the absence of grazers leading to the establishment of high algal standing stocks in platelet layers (e.g. Smetacek and others, Reference Smetacek1992). In contrast, other studies have recorded high numbers of protozoan and metazoan grazers in platelet layers (Arrigo and others, Reference Arrigo1995; Archer and others, Reference Archer, Leakey, Burkill, Sleigh and Appleby1996; Grossmann and others, Reference Grossmann, Lochte and Scharek1996; Günther and others, Reference Günther, George and Gleitz1999a, Reference Günther, Gleitz and Dieckmann1999b). As with the bottom sea-ice communities, the sub-ice platelet layer communities provide an important nutritional resource for invertebrates such as juvenile krill (Marschall, Reference Marschall1988; Frazer, Reference Frazer1996; Quetin and others, Reference Quetin, Ross, Frazer and Haberman2013), amphipods (Rakusa-Suszczewski, Reference Rakusa-Suszczewski1972) and other zooplankton. Much of their production is exported to deeper layers by means of rapidly sinking faecal pellets of copepods and krill (Gonzales, Reference Gonzales1992; Daly, Reference Daly2013; Schnack-Schiel and others, Reference Schnack-Schiel, Thomas, Dahms, Hass and Mizdalski2013).
7.5.3 Fish
The notothenioid Antarctic silverfish (Pleuragramma antarcticum) has a pivotal importance in Southern Ocean ecosystems. In the adult stage, this fish is widely distributed and abundant in shelf waters around the continent, and represents a major contribution to the diet of most Antarctic vertebrates such as whales, seals, penguins, flying birds and benthic fish, especially in the Ross Sea (La Mesa and others, Reference La Mesa, Eastman and Vacchi2004). They also constitute the major link between lower (invertebrates) and higher (birds and mammals) levels of the food web. The sub-ice platelet layer has been found to play a crucial role in the early stages of the life cycle of this species (Vacchi and others, Reference Vacchi, La Mesa, Dalu and Macdonald2004), providing an important food source, but also a favourable environment, protected from predation (Gutt, Reference Gutt2002). These fish use antioxidants as adaptive responses to extreme environmental conditions and to the rapid changes of pro-oxidant pressure associated with the sub-ice platelet layer (Regoli and others, Reference Regoli, Nigro, Benedetti, Fattorini and Gorbi2005). It is one of the major aims of the MOO (Cziko, Reference Cziko2019) to support studies on the freezing-avoidance abilities of Antarctic notothenioid fishes.
7.5.4 Mammals and birds
The exact roles and implications of platelet ice for higher trophic levels are still unclear. There are, to our knowledge, no studies that explicitly investigate the importance of the platelet-ice realm for seals or penguins. However, fast ice extent, stability and timing of break-up is a critical factor for Emperor Penguin adult survival in winter (Barbraud and Weimerskirch, Reference Barbraud and Weimerskirch2001; Jenouvrier and others, Reference Jenouvrier, Barbraud and Weimerskirch2005), breeding success (e.g. Massom and others, Reference Massom2009; Trathan and others, Reference Trathan, Fretwell and Stonehouse2011) and moult (Croxall and others, Reference Croxall, Trathan and Murphy2002). Furthermore, Emperor penguins mainly feed on Antarctic krill Euphausia superba and Antarctic silverfish Pleuragramma antarcticum. The prey composition suggests that these penguins perform shallow dives to explore the underside of sea ice where this food is foraged (Klages, Reference Klages1989). Since fish and other small animals that mammals and birds rely on as a food source thrive in sub-ice platelet layers for the above reasons, one can hypothesise that this is why the mammals and birds tend to live close to these regions. This is especially true for the summer, during which melting platelet ice releases masses of algae and other organic particles into surface layers, creating locally attractive feeding spots. This results in an intensive foraging in zooplankton, krill and fish which aggregate under the melting sea ice, and which in turn attracts the seals (Plötz and others, Reference Plötz, Bornemann, Knust, Schröder and Bester2001). Since the additional thickness provided by incorporated platelet ice contributes to the stability of coastal fast-ice regimes, and also because there is an increased supply of food due to the presence of a sub-ice platelet layer, it is likely that these conditions favour the breeding of Emperor penguins at those sites.
One particularly interesting adaptation of seals to sub-ice platelet layers was found by Davis and others (Reference Davis1999). Using a seal-borne camera, they showed how a seal was blowing bubbles to flush a fish (Pagothenia borchgrevinki) out of the platelet layer (see Figs 2j and k). They also saw how seals, in addition to biting exposed fish tails and flushing the fish out, may even pursue the fish into the sub-ice platelet layer.
8. Outlook
How will the properties of platelet ice change in a future higher greenhouse gas world? The overly-simplistic conceptual model ‘warmer ocean leads to more basal melting leads to more platelet ice’ is likely not applicable in such a complex system. ISW is a necessary but not sufficient precursor for platelet-ice formation. Furthermore, ISW presence and circulation in a future Southern Ocean is likely to be different in the Ross Sea, Weddell Sea and other regions where platelet ice is presently observed. HSSW formation in polynyas is the most important precursor for ISW formation in cold cavity ice shelves where Mode 1 melting dominates (Jacobs and others, Reference Jacobs, Hellmer, Doake, Jenkins and Frolich1992). Spatial and temporal changes to katabatic winds, and therefore changes to HSSW production in polynyas, would thus lead to changes in Mode 1 generated ISW and platelet-ice formation.
Stronger wind events in a future climate could on the one hand enhance sea-ice formation in polynyas and lead to greater HSSW production, and thus accelerate the circulation in the cavity. Subsequent heat advected to the cavity would cause stronger Mode 1 melting at the deep ice-shelf base, and eventually increase ISW and platelet-ice formation. Although this argument holds true for polynyas linked to katabatic winds which will retain sub-freezing temperatures (but may vary in occurrence frequency and event duration) even with a warming climate, overall expected warmer Antarctic winters allowing less sea-ice production could potentially cause the opposite. Less HSSW production will slow down the cavity circulation and the associated heat influx, thus reducing Mode 1 ISW production (Nicholls, Reference Nicholls1997). Although speculative, less and slower outflow of ISW could potentially generate a thicker layer of supercooled water, and, by maintaining the heat sink for longer, give frazil crystals more time to form. Thus, less HSSW production could actually contribute to frazil ice generation, even if the frazil crystals might never make it to the front. Instead, they are deposited at the ice-shelf base as marine ice.
On the other hand, stronger wind events (especially when paired with a reduced sea-ice cover) cause stronger lateral mixing in the upper ocean, thus eroding horizontal density gradients which drive the ocean circulation over the continental shelf (Jendersie and others, Reference Jendersie, Williams, Langhorne and Robertson2018). Even with potentially warmer and more accessible CDW, a slowed circulation between ice shelves and the deep Southern Ocean would transport less oceanic heat sourced from CDW towards the ice-shelf cavity, possibly reducing Mode 2 melting (Jendersie and others, Reference Jendersie, Williams, Langhorne and Robertson2018).
An observed increase in wind speeds over the ACC over the past five decades (Marshall, Reference Marshall2003) might be linked to the poleward shift of southern hemisphere Westerlies caused by global warming (Russell and others, Reference Russell, Dixon, Gnanadesikan, Stouffer and Toggweiler2006). The resulting increased overturning transport within the ACC through enhanced eddy activity (Hallberg and Gnanadesikan, Reference Hallberg and Gnanadesikan2006; Meredith and Hogg, Reference Meredith and Hogg2006; Hogg and others, Reference Hogg, Meredith, Blundell and Wilson2008; Meredith and others, Reference Meredith, Naveira Garabato, Hogg and Farneti2012) has possibly made CDW more available to some parts of the Antarctic continental shelf already (Thoma and others, Reference Thoma, Jenkins, Holland and Jacobs2008; Dinniman and others, Reference Dinniman, Klinck and Hofmann2012; Gille and others, Reference Gille, McKee and Martinson2016).
Its wind-related shallower residence may contribute to the growing CDW-sourced heat exposure and melting of ice shelves along the West Antarctic Peninsula seen today (Rignot, Reference Rignot2008; Jenkins and others, Reference Jenkins2010; Dinniman and others, Reference Dinniman, Klinck and Smith2011, Reference Dinniman, Klinck and Hofmann2012). Timmermann and Hellmer (Reference Timmermann and Hellmer2013) predict that increased CDW transport to the grounding line of the Filchner–Ronne Ice Shelf will lead to four to six times higher basal mass loss by the next century, potentially making the occurrence of platelet ice less likely. For the Ross Sea on the other hand, simulations suggest a reduction in on-shelf transport of CDW over the next 100 years (Smith and others, Reference Smith, Dinniman, Hofmann and Klinck2014), thus decreasing potential Mode 2 melting. Therefore, the modification of the Ross Ice Shelf from a cold-cavity to a warm-cavity ice shelf is unlikely over the next 100 years. Platelet-ice formation is therefore still likely to occur in this region, which includes the McMurdo Ice Shelf, on that timescale.
Other conceivable climate change related impacts on future Modes 1 and 3 melting and subsequent platelet-ice formation are the observed freshening in the Ross Sea (Jacobs and others, Reference Jacobs, Giulivi and Mele2002; Assmann and Timmermann, Reference Assmann and Timmermann2005; Jacobs and Giulivi, Reference Jacobs and Giulivi2010), and a possible strengthening of the stratification of the upper water column through a fresher and warmer summer surface mixed layer. Lower salinity HSSW may decrease the deep basal melting under ice shelves (Hellmer and Jacobs, Reference Hellmer and Jacobs1995) thus slowing the local thermohaline overturning circulation. A prolonged surface freshwater layer may prevent ISW from rising to shallower depths and reaching in situ supercooling state. Conversely, fresher surface water with a higher freezing temperature facilitates earlier sea-ice formation, HSSW production and deep convection, which in turn decreases the stratification.
Clearly, with the multitude of mutually compensating processes mentioned here, more research is needed to detail and quantify the complex interaction between the various mechanisms involved, which govern the formation and melting of sea ice and platelet ice, heat advection into ice-shelf cavities, and the evolution of CDW access to ice shelves under global warming conditions.
9. Conclusions
First, our literature study shows that there seems to be a substantial body of research available that is associated with platelet ice in one way or another, certainly more than one would see at the first glance when, for example, conducting a keyword search on the common scientific portals. The knowledge is, however, extremely scattered, partly because the terminology has never been clearly defined. This paper tries to change that, by making authors of future studies aware of the issue and proposing terminology which should be used in the most consistent way possible, and that terminology is being adopted by the next update of JCOMM Expert Team on Sea Ice (2015).
Second, we conclude that the formation and presence of platelet ice, especially in its semi-consolidated form (sub-ice platelet layer), plays an exceptionally important role in a number of regions of coastal Antarctica that are associated with cold ice-shelf cavities. In an attempt to illustrate the far-reaching impacts for those regions, we compiled a scheme that summarises all the different aspects which have been touched upon in this paper (Fig. 6).

Fig. 6. Overview of origin (left) and implications (right) of platelet ice. Several aspects related to the role of incorporated platelet ice on the right also apply more generally to fast ice itself (see also Fraser and others, Reference Fraser, Massom, Michael, Galton-Fenzi and Lieser2012), with the presence of platelet ice further enhancing these effects.
Third, most of the current knowledge and understanding of the processes related to platelet ice stems from observations in key areas like the Ross and eastern Weddell Seas, owing to only a small number of dedicated research programmes. Of the existing fast-ice and platelet-ice datasets, McMurdo Sound clearly stands out with its more than 100 year history of observations (Langhorne and others, Reference Langhorne2015). In a few other regions, monitoring programmes have been established more recently, such as in Atka Bay (Arndt and others, Reference Arndt, Hoppmann, Schmithüsen, Fraser and Nicolaus2020), and near Rektangelbukta (personal communication from Gerland and others, 2019).
Ocean–ice shelf interaction has become an important topic in polar research in recent years, and many initiatives were founded for this purpose or have already incorporated relevant elements into their research programmes (e.g. the Forum for Research into Ice Shelf Processes FRISP; the Southern Ocean Observing System SOOS, Rintoul and others, Reference Rintoul2011; Observing and Understanding the Ocean below Antarctic Sea Ice and Ice Shelves OASIIS, Rintoul and others, Reference Rintoul2014; the Antarctic Fast Ice Network, Heil and others, Reference Heil, Gerland and Granskog2011; the Ross Ice Shelf Programme (Antarctica New Zealand) and many more). More engagement from these networks is however necessary to better guide and coordinate the international efforts with regards to fast ice and platelet ice. This could be done by providing best practice and standard operating procedures to help establish more monitoring sites at wintering stations, as well as to collect and maintain a database of all the scattered published and particularly the unpublished observations.
Fourth, only integrated, multidisciplinary research programmes are able to advance our knowledge of the many platelet-ice processes and their complicated linkages beyond the properties of the physical system. Therefore, we recommend that any new research project on the platelet-ice system be designed on a multidisciplinary basis, even though this can be challenging. Such a project needs to include process studies and long-term monitoring alike.
Fifth, to achieve a more complete and comprehensive picture of its physical properties, distribution, seasonal evolution and the associated biological processes and biogeochemical cycles, the observational techniques related to platelet ice have to be improved. There is some hope that recently developed technologies such as electronmagnetic sensing methods and improved thermistor chains, as well as the application of research platforms such as aircraft, ROVs and AUVs will be able to provide this. There is still a long way to go until we are able to study platelet ice with a much more integrated approach, in much greater detail and on much larger scales than currently feasible. Another gap is the lack of interaction between the observational and numerical studies, which currently do not provide enough feedback between each other. As pointed out in our third conclusion above, although there are initiatives and networks that are gathering observations on platelet-ice processes, there is not an over-arching coordination of efforts with regards to procedures, winter data gathering or data management. This lack of communication hinders analysis and comparison of data, especially unpublished or hard-to-find historical data. Long-term monitoring is difficult to obtain support for in most national Antarctic programmes, yet is critical for evaluating changes in systems such as ocean–ice-shelf systems. Very few research groups or projects related to platelet ice include observationalists, process modellers and larger-scale (e.g. climate and ocean–ice shelf–sea ice) modellers. These kinds of close collaborations need to be resourced at an appropriate level of time, money and logistics to be able to ensure the following occur: ground-truthing models against observations; having enough observations to constrain models; and knowing whether or not the models are missing components/physics that have been observed in the field. Modellers, for example, would like to know how common platelet ice is and how much of an effect it will have on climate-scale results. Observationalists, on the other hand, would like to know where ocean–ice shelf–sea ice models predict platelet ice will occur and therefore where observations should be carried out to test these predictions. A significant gap also exists with regards to ecological modelling of the platelet layer system, which has so far not extended beyond the skeletal layer at the sea ice underside (Arrigo and others, Reference Arrigo, Kremer and Sullivan1993a).
Sixth, it is extremely difficult to make accurate general statements about the implications of climate change on platelet-ice processes that are more than just speculation or educated guesses. Although so far no significant changes in the occurrence and seasonality of platelet ice have been observed, at least in the Ross and Eastern Weddell Seas (Langhorne and others, Reference Langhorne2015; Arndt and others, 2020, respectively), that might not be the case in so far unmonitored locations. It is therefore crucially important that we tackle these issues sooner rather than later, before the significant changes related to the changing climate, as currently observed in West Antarctica and several of the East Antarctic glaciers, spread further.
Finally, we can safely say that the realms of sub-ice platelet layers are one of the least explored and at the same time highly productive ecosystems on our planet, inhabited by a multitude of yet undiscovered (micro-) organisms. We urgently need to improve our understanding of these systems and the complex inter-linkages with their environment to better advise stakeholders and decision makers how to preserve them.
Acknowledgements
MH was supported by the German Research Council in the framework of the priority programme ‘Antarctic Research with comparative investigations in Arctic ice areas’ SPP1158 under grant number NI 1092/2. IJS and PJL thank the following funding agencies for support: University of Otago research grants 110282, 112061 and 110312; Antarctica New Zealand and the Scott Base staff for providing essential field and logistical support; the Deep South National Science Challenge for projects ‘Targeted Observations and Processes Informed by Modelling of Antarctic Sea Ice’ and ‘Impacts of freshwater from icebergs and ice shelf melt in the New Zealand Earth System Model’; a subcontract to National Institute of Water and Atmospheric Research (NIWA) Core funding ‘Antarctic and High Latitude Climate’. MH acknowledges Jean-Louis Tison (Université Libre de Bruxelles), Marcel Nicolaus, Hauke Flores, Hartmut Hellmer, Ralph Timmermann and Horst Bornemann (AWI) for the fruitful discussions. IJS and PJL are grateful to the following people for on-going discussions on platelet ice and McMurdo Sound sea ice that helped inform this paper: Greg Leonard (University of Otago), Joe Trodahl (Victoria University of Wellington), Daniel Pringle, Jeff Scanniello (United States Antarctic Program Survey Team), Mike Williams (NIWA), and Craig Stevens (NIWA and the University of Auckland), Natalie Robinson (NIWA), Alex Gough, Andy Mahoney (University of Alaska Fairbanks), Tim Haskell, Pat Wongpan and Ken Hughes. PJL acknowledges: FRST funding through contract IPY UOOX0705, and by subcontracts to NIWA; project C01X1226 funded by Ministry of Business, Innovation and Employment; and numerous core funding projects, most recently CAOA1703; support and hospitality of the Isaac Newton Institute for Mathematical Sciences, Cambridge during the Mathematics of Sea Ice Phenomena (supported by EPSRC grant no EP/K032208/1). IJS was supported by the 1999/2000 Antarctica New Zealand Sir Robin Irvine Post-Graduate Scholarship, the University of Otago and a Blair Trust scholarship; the Aotearoa New Zealand Ross Ice Shelf Programme: Vulnerability of the Ross Ice Shelf in a Warming World (funded by the New Zealand Antarctic Research Institute, grant number: NZARI 2014-11, PI: Christina Hulbe). SJ was supported through the NZ SeaRise programme, funded by the Ministry of Business, Innovation and Employment. MER is grateful to Christian Haas and the Sea Ice Physics Section at AWI for a student grant. The authors acknowledge the JCOMM Expert Team on Sea Ice for their support in including the relevant terminology in the official Sea Ice Nomenclature. The authors are most grateful to two anonymous reviewers for their valuable comments which helped to further improve the manuscript. Finally, we acknowledge support by the Open Access Publication Funds of Alfred-Wegener-Institut Helmholtz-Zentrum für Polar- und Meeresforschung.
Author contributions
All authors contributed significant time and effort to the writing of this paper. DNT and GSD had the initial idea and started the writing process. PJL and IJS carried out further drafting of the paper and expanded the list of papers reviewed. MH and MER then re-structured and re-wrote the entire manuscript, while IJS, PJL and SJ contributed to several sections and provided extensive feedback. All authors have contributed to final editing and given their approval for submission.