There is substantial evidence suggesting that the perinatal nutritional environment has profound implications for the development and long-term health outcomes in the offspring( Reference Barker 1 – Reference O’Sullivan, Little and Combes 3 ). This may be a consequence of ‘fetal programming’, a phenomenon that describes the adaptive responses of the offspring to the environmental cues during the initial phases of life( Reference Barker 1 , Reference Godfrey and Barker 2 ).
Fetal metabolic programming occurs because of the modulation of gene expression mediated by epigenetic mechanisms that can permanently affect the structure and functions of tissues and organs, changing the number, distribution and differentiation of different cell types( Reference O’Sullivan, Little and Combes 3 ). The higher plasticity rate and sensibility to the environmental changes generate a unique genetic opportunity, which can influence the phenotype( Reference Portha, Fournier and Ah Kioon 4 ). Since the past decades, a plethora of epidemiological and experimental evidence supports the allegation that fetal and early postnatal timings, compared with other phases of life, are paramount to establish the susceptibility for non-communicable chronic diseases such as obesity( Reference Huda, Brodie and Sattar 5 , Reference Jones and Friedman 6 ).
Therefore, the early-onset and increased rates of obesity seen nowadays should not be attributed to genetic and/or environmental factors alone. It is well known that obesity has a multifactorial and complex aetiology, which derives from an energy imbalance – when energy intake is greater than energy expenditure. Obesity is defined by adipose tissue storage and white adipose tissue (WAT) expansion that occurs through adipocyte hypertrophy and hyperplasia( Reference Siriwardhana, Kalupahana and Cekanova 7 ). WAT is recognised by its secretory capacity and role in the energy homoeostasis for storing TAG when energy exceeds or releasing free fatty acids (FFA) when there is an energy deficit( Reference Lehr, Hartwig and Lamers 8 ). On the other hand, brown adipose tissue (BAT) is recognised by its thermogenic function, but also plays a role in the energy homoeostasis and could contribute to obesity control( Reference Kim and Plutzky 9 ).
Obesity, considered a pandemic disease, has more than doubled since 1980. It is one of the greatest challenges of public health care in the world( 10 ). The increased consumption of red meats, refined cereals, industrialised and fried foods, which are characteristics of a Western diet, low in fibre, high in fat and carbohydrates of poor quality, contributes to increasing these indices( Reference Fleming, Robinson and Thomson 11 ).
Diet, besides its impact on health and disease, can also influence the offspring’s phenotype, depending on what the mother ate during gestation and lactation periods( Reference Barker 1 – Reference O’Sullivan, Little and Combes 3 ). Experimental models showed that it is expected to find phenotypes of body weight loss in the neonate and obesity and cardiometabolic risk in the offspring whose maternal diets were poor in proteins( Reference Claycombe, Vomhof-DeKrey and Roemmich 12 ), rich in fats( Reference Bruce and Jonscher 13 ) and Western diet style( Reference Szostak-Wegierek 14 ), respectively. A similar process occurs between diet and BAT: diet can stimulate( Reference Rippe, Berger and Böiers 15 ) and programme the development of this tissue, as it can act directly on the uncoupling protein 1 (UCP-1)( Reference Felipe, Villarroya and Mampel 16 ). The epigenetic mechanisms are possibly the main explanation for the potential evolutionary reasons that offspring BAT might be programmed by maternal diet( Reference Chen, Buyel and Hanssen 17 ). Researchers revealed that foods typical of a Western diet – for instance, margarine – were related to impaired BAT function and UCP-1 activation in experimental models of fetal programming( Reference Priego, Sánchez and García 18 ); similar outcomes were found in adult animals( Reference Fromme and Klingenspor 19 ).
In BAT, the mitochondrial inner membrane holds UCP-1, which is the main factor responsible for thermogenesis. The mechanism of action of UCP-1 is to uncouple oxidative phosphorylation from an ADP molecule, resulting in greater oxidation of substrates, and heat production( Reference van Marken Lichtenbelt and Schrauwen 20 ). During cellular respiration, the uncoupling of oxidative phosphorylation represents 20–50 % of all energy expended by the mitochondria of a normal functioning cell( Reference Rolfe, Newman and Buckingham 21 ). Under conditions of controlled cold exposure, estimates are that 60 g of BAT could contribute to up to 20 % of heat production in humans( Reference Ouellet, Labbé and Blondin 22 ).
Seemingly, there exists a direct relation among balanced diet, UCP-1 metabolism and fetal BAT programming, as well as a potential connection between an inadequate diet and impaired BAT function, which may be correlated with the onset of obesity. Therefore, we question what might be the real action of the fatty acids on the BAT. Also, we wonder whether different fatty acids might modulate BAT and UCP-1 differently and how strong is the evidence that fatty acids from the maternal diet could programme BAT in experimental models. In addition, research investments on fetal programming concerning BAT may represent a relevant way to tackle obesity and its comorbidities. Therefore, this review brings to light the relation of fetal programming mediated by fatty acids on the offspring BAT.
Methods
We have selected published manuscripts from 1988 to 2018, in the English language, using a combination of the following index terms: brown adipose tissue, uncoupling protein 1, fetal programming and fatty acids. Five databases of published literature were used: PubMed, Cochrane Library, EMBASE, Medline and ScienceDirect.
Brown adipose tissue
It was once believed that BAT was only present in hibernating animals, rodents and newborns( Reference Clarke, Bryant and Lomax 23 ). The interest in BAT grew after studies found it in adult humans, which inversely correlated with BMI( Reference Vijgen, Bouvy and Teule 24 ). Increased BAT and UCP-1 activity are also related to glucose and lipid metabolism improvement( Reference Kim and Plutzky 9 , Reference Bartelt, Bruns and Reimer 25 ). In newborns, BAT is responsible for non-shivering thermogenesis, assuring an efficient adaptation to the environment, preventing hypothermia and possibly neonatal death( Reference Clarke, Bryant and Lomax 23 ). Consequently, the uncoupling oxidative phosphorylation process is paramount in the regulation of energy balance in many developmental phases (Fig. 1).
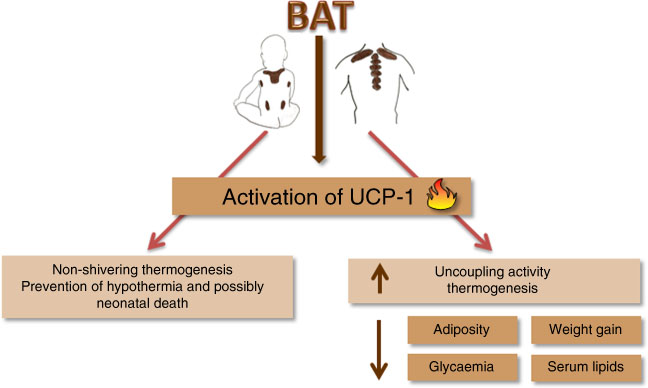
Fig. 1 Mechanism of action of uncoupling for the prevention of neonatal death and metabolic adult diseases. BAT, brown adipose tissue; UCP-1, uncoupling protein 1.
Uncoupling protein-1 activation rate in BAT depots vary according to the fatty acid availability and flow into the cells( Reference Ricquier and Bouillaud 26 ). For this reason, there is a potential for dietary fatty acids to act on the fetal BAT and UCP-1 activation. It is acknowledged that BAT depots and UCP-1 differ between species and with age( Reference Symonds, Dellschaft and Pope 27 , Reference Hayman, Sciences and Boston 28 ). There are differences in the main BAT depots at birth between different animal species. In rats, BAT is found mainly in the interscapular region and small quantities at birth, which reaches its capacity to produce heat only after birth( Reference Symonds, Pope and Sharkey 29 ). In human babies, there are huge BAT depots, and these are located in the supraclavicular and neck, pericardium and perirenal regions. Moreover, they also have an axial BAT depot( Reference Symonds, Pope and Budge 30 ).
Distinct from WAT, BAT is characterised by multilocular lipid droplet phenotype, higher mitochondrial density and lower secretory activity. Regarding cell lineage, brown pre-adipocytes derive from cellular precursors that express encoding myogenic factor 5, a gene expressed in myogenic cell lineage( Reference Bartelt and Heeren 31 ). Despite their differences in function and development, they have in common the primary adipogenesis regulators, which are transcriptional factors such as peroxisome proliferator-activated receptor (PPAR) and CCAAT-enhancer binding proteins (C/EBPp)( Reference Seale, Bjork and Yang 32 ).
Regulation of brown adipose tissue thermogenesis by dietary fatty acids
Brown adipose tissue is highly vascularised and innervated by the sympathetic nervous system, and its response varies depending on the nature of the external stimulus, such as age, sex, genetics and also diet( Reference Cypess, Lehman and Williams 33 ). One of the first works published in 1988 showed that 48 h of fasting reduced interscapular BAT depots and UCP-1 gene expression, and lowered the thermogenic capacity in adult mice. Moreover, a refeeding period of 10–15 d was enough to re-establish normal thermogenic capacity in the animals, highlighting a pivotal role of the diet in the functioning of this tissue and the activation of UCP-1( Reference Trayhurn and Jennings 34 ).
Despite diet-induced thermogenesis being a controversial subject in the literature( Reference Kozak 35 ), there is not yet a consensus about which nutritional cue may activate UCP-1 in BAT. However, vitamin A( Reference Kumar, Sunvold and Scarpace 36 – Reference Felipe, Bonet and Ribot 41 ), some amino acids such as l-arginine( Reference Jobgen, Meininger and Jobgen 42 ) and l-ornithine( Reference Konishi, Koosaka and Maruyama 43 ), some bioactive compounds such as anthocyanins( Reference Kanamoto, Yamashita and Nanba 44 ), resveratrol( Reference Andrade, Frade and Guimarães 45 – Reference Ku, Cho and Hong 47 ), isoflavones( Reference Crespillo, Alonso and Vida 48 , Reference Kamiya, Nagamine and Sameshima-Kamiya 49 ), catechins( Reference Nirengi, Amagasa and Homma 50 – Reference Kudo, Arai and Suhara 52 ) and flavonoids( Reference Matsumura, Nakagawa and Mikome 53 ) may stimulate UCP-1 in adult rats BAT. Because fatty acids sustain lipid oxidation and act as uncouplers of mitochondrial oxidative phosphorylation, thus increasing thermogenesis( Reference Smith, Carstens and Randel 54 , Reference Sellayah, Bharaj and Sikder 55 ), a plethora of experimental model studies are now investigating how dietary fats activate UCP-1 in BAT.
Authors have shown positive regulation of UCP-1 mRNA and protein levels in the BAT of adult rats and young mice, after a high-fat diet intake( Reference Fromme and Klingenspor 19 , Reference Cannon and Nedergaard 57 , Reference Ho, Calingasan and Wille 58 ). However, higher UCP-1 gene expression not always means lower adiposity and weight gain( Reference Jeyakumar, Vajreswari and Giridharan 37 , Reference Felipe, Bonet and Ribot 41 , Reference Liang, Yang and Zhang 59 ). A recent study showed that a high-fat diet during lactation causes increased weight gain, body fat depots and UCP-1 gene expression in offspring BAT after weaning( Reference Liang, Yang and Zhang 59 ). In addition, the transcription of other genes involved in the thermogenesis, such as positive regulatory domain containing 16 (PRDM16), PPARγ coactivator 1α (PGC-1α), cell-death-inducing DNA fragmentation factor A-like effector A (CIDEA), cytochrome c oxidase subunit 7-α (COX7) and elongation of very-long-chain fatty acids like 3 (ELOVL3) was unaltered in BAT. Moreover, when these animals were exposed to cold, they showed reduced UCP-1 gene expression, suggesting a decline in the cold-induced adaptive thermogenesis. During adulthood, they showed reduced UCP-1 gene expression and the above-cited thermogenesis markers in BAT( Reference Liang, Yang and Zhang 59 ). The authors concluded that a high-fat diet damaged the thermogenesis in BAT, and it has been associated with persistent adiposity and the development of metabolic syndrome in adulthood( Reference Liang, Yang and Zhang 59 ). Therefore, evidence indicates that high lipid levels could propitiate a momentary increase of UCP-1 in the offspring, albeit it does not overcome the adiposity that this diet can cause( Reference Oi-Kano, Kawada and Watanabe 60 ).
Supplementation with 30 % of olive oil, rich in MUFA, for 28 d reduced body weight and weight gain, and increased UCP-1 gene expression in BAT of 7-month-old male Sprague–Dawley rats( Reference Rodriguez, Portillo and Pico 61 ). Other scientists fed adult male Wistar rats having an average body weight of 240 (sd 2) g with 40 % of total energy from olive oil for 4 weeks, and they have not found body weight alteration, but as already seen by other studies they found increased UCP-1 mRNA in the interscapular BAT( Reference Vögler, Ló Pez-Bellan and Alemany 62 ). Besides, oral supplementation with oleic and 2-hydroxyoleic acids and long-chain MUFA C18 (600 mg/kg), every 12 h for 7 d, did not change UCP-1 gene expression in BAT but caused weight loss in 16-week-old male Wistar Kyoto rats. The absence of changes in UCP-1 gene expression in BAT could be related to the short-term exposure to treatment( Reference Clarke 63 ). Hence, despite the time of diet exposure being an important factor, these studies confirm that the type and amount of fatty acids prompt UCP-1 gene expression in BAT.
In the literature, PUFA, especially the n-3 family, could stimulate thermogenesis, lipid metabolism and lead to weight loss( Reference Kunešová, Hlavatý and Tvrzická 64 ). DHA (22 : 6n-3), a type of n-3 PUFA, is associated with weight loss in obese women( Reference Mori, Bao and Burke 65 ). A similar outcome was seen in overweight mice that consumed one daily portion of fish for 16 weeks( Reference Bargut, Silva-e-Silva and Souza-Mello 66 ). A 3-month-old male C57BL/6 mice supplemented with 119 and 238 g/kg of fish oil containing EPA and DHADHA for 8 weeks improved metabolic profile and positively affected UCP-1 mRNA and protein expression in BAT. In the same study, increased thermogenesis positively correlated with lower weight gain( Reference Bargut, Frantz and Mandarim-De-Lacerda 67 ). However, n-3 PUFA may lower the expression of lipogenic proteins such as acetyl-CoA carboxylase, fatty acid synthase and malonyl-CoA and raise protein expression of carnitine palmitoyltransferase 1. The latter is responsible for fatty acid translocation from the cytoplasm to the mitochondria, favouring β-oxidation, which in turn can contribute to thermogenesis( Reference Suchankova, Tekle and Saha 68 ). Although the literature still requires more insights into the mechanisms of action of different fatty acids on BAT, UCP-1 and lipogenic proteins, considerable advances have been made in the identification of the transcriptional factors and co-regulatory proteins promoting embryological development and the acquisition of thermogenic profile in BAT. Among the transcriptional factors, highlight the PPAR, even though UCP-1 could be induced in their absence, PPAR are related to diet and fatty acids( Reference Rodríguez-Cruz and Serna 69 ). PPARα and PPARγ are regulated by PGC-1α ( Reference Hondares, Rosell and Díaz-Delfín 70 , Reference Festuccia, Blanchard and Richard 71 ). PGC-1α is a transcriptional coactivator that has a critical role in the activation of cAMP-dependent protein kinase A, the protein responsible for lipolysis and thermogenesis in BAT, which responds to cold and diet triggers via β-adrenergic receptors under norepinephrine control( Reference Cao, Daniel and Robidoux 72 ). Another gene highly expressed in BAT, and regulated by PPARα, is PRDM16. PPARα can induce gene transcription of PGC-1α in brown adipocytes (BA) through mechanisms involving PRDM16. Thus, PPARα regulates thermogenesis in brown fat by inducing gene expression of PGC-1α and PRDM16, which leads to higher UCP-1 expression and mitochondrial oxidative activity( Reference Hondares, Rosell and Díaz-Delfín 70 ). Other proteins such as CIDEA can control thermogenesis by inducing UCP-1 expression( Reference Zhao and Chen 73 ). Also, a protein product of fibroblast growth factor 21 (FGF21) may act as a potential adipogenic adipokine, which influences thermogenesis by upregulating UCP-1 expression in progenitors isolated from human cervical fat differentiated into BA-like( Reference Lee, Werner and Kebebew 74 ). There are also the sirtuin 1 (SIRT1) and sirtuin 3 (SIRT3), protein family of seven histone deacetylases (class III), that function as redox sensors that respond to changes in NAD/NADH levels. The stimulation of SIRT1 and SIRT3 may lead to an increased UCP-1 expression in BAT( Reference Pahlavani, Razafimanjato and Ramalingam 75 ). SIRT3 is also positively regulated by PGC-1α ( Reference Waldén, Hansen, Timmons, Cannon and Nedergaard 76 ), increasing UCP-1 and mitochondrial gene expression in this tissue( Reference Pahlavani, Razafimanjato and Ramalingam 75 , Reference Waldén, Hansen, Timmons, Cannon and Nedergaard 76 ). Published research shows that these proteins could be regulated by fatty acids, in particular EPA( Reference Zhao and Chen 73 , Reference Pahlavani, Razafimanjato and Ramalingam 75 , Reference Qin, Zhou and Chen 77 ). Additionally, the presence of G-protein-coupled receptor 120 (GPR120, also known as FFA receptor 4 (FFAR4)), a cellular receptor for PUFA in the BA, may cooperate in the regulation of the transcriptional factors. Corroborating this view, a recent study found that the activation of GPR120 induces the release of FGF21 by BA( Reference Quesada-López, Cereijo and Turatsinze 78 ). Hence, EPA may activate some transcriptional factors, such as PPAR, in the BA nucleus. In turn, it causes an increased UCP-1 gene expression and boosts BA activity, thus leading to accretion in the energy expenditure.
Epigenetic mechanisms are involved with UCP-1 and thermogenesis( Reference Chen, Buyel and Hanssen 17 , Reference Shore, Karamitri and Kemp 79 ). These are responsible for the formation, maintenance and reversion of gene transcriptional patterns. The main epigenetic events include DNA methylation, histone post-translational modifications (acetylation, methylation, phosphorylation and others) and non-coding RNA( Reference Seki, Williams and Vuguin 80 ). Damped DNA methylation in the promoter region of the UCP-1 gene was inversely related to the expression of this protein( Reference Shore, Karamitri and Kemp 79 ). Histone acetylation is involved in gene transcriptional control of thermogenesis in BAT( Reference Shi, Wang and Stieren 81 ). In this context, class 1 and class 3 of sirtuin deacetylases (SIRT1 and SIRT3) altered the thermogenic function by deacetylation of PGC-1α. In contrast with the thermogenic function activated by the inhibition of histone deacetylases, in this same study, the overexpression of SIRT1 in 2-month-old mice increased BAT activity, leading to a greater energy expenditure( Reference Boutant, Joffraud and Kulkarni 82 ). Demethylases were also found to be involved in the differentiation process of BA in mice BAT( Reference Zha, Li and Wu 83 ). Recently, it has been shown that BA release exosomes, and the activation of BAT increases their release( Reference Seki, Williams and Vuguin 80 ). MicroRNA are a subclass of non-coding RNA that regulate protein expression( Reference Pfeifer and Lehmann 84 ). The literature conveys that they may be implicated in the functioning of this tissue( Reference Trajkovski and Lodish 85 ). In adult humans, miR-92a exosome levels were inversely correlated with BAT activity, which was measured by positron emission tomography with F-18 fluorodeoxyglucose (18F-FDG PET/CT). In this study, microRNA was considered a potential human serum biomarker of BAT activity( Reference Chen, Buyel and Hanssen 17 ). It has been revealed that EPA is related to increased gene expression of promoters of brown fat development, as well as UCP-1, through mechanisms that entail microRNA modulation in subcutaneous pre-adipocytes of women with a BMI range of 28.1–29.8 kg/m2 ( Reference Laiglesia, Lorente-Cebrián and Prieto-Hontoria 86 ). A study with palmitic acid, oleic acid and EPA found that the latter (100 mm) was associated with microRNA clusters at inducing the activation of the FFAR4, which is also known as GPR120, in primary brown cells. Later, this signalling axis (EPA/FFAR4/microRNAs) was confirmed in C57BL/6 adult male mice fed fish oil (15 %) for 12 weeks( Reference Kim, Okla and Erickson 87 ).
There is still a lot to discover about the epigenetic mechanisms in BAT and UCP-1 expression, and even more about their regulation mediated by different fatty acids in the diet. In spite of this, at the moment, the literature has shown that the mechanisms involving fatty acids, especially EPA, beta-oxidation enzymes, thermogenic transcriptional factors, specific receptors and other thermogenic proteins, seem to be interconnected to regulate and activate UCP-1 and thermogenesis in BAT. Therefore, they could be a plausible target to increase energy expenditure and aid in the treatment of obesity (Fig. 2).
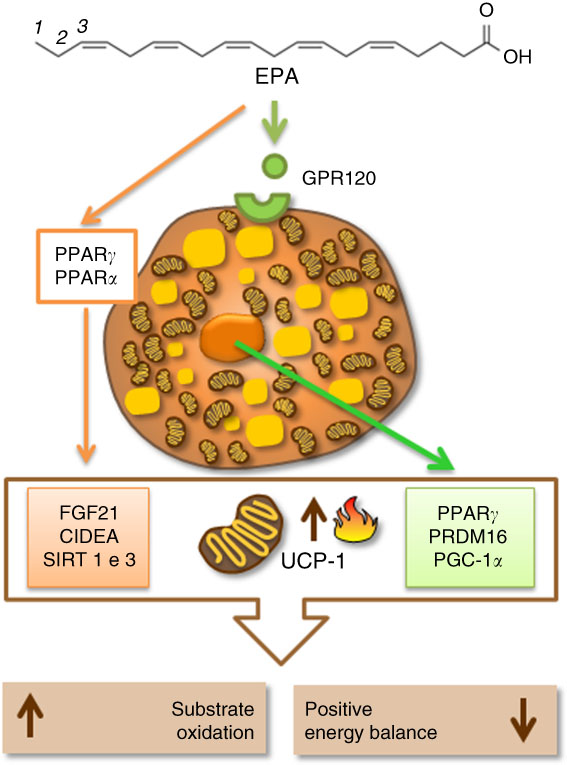
Fig. 2 Eicosapentaenoic acid regulating thermogenesis and uncoupling protein 1 (UCP-1) expression by activation of specific cellular receptors (G-protein-coupled receptor 120 (GPR120)) in the brown adipocyte or by activation of proteins related to fatty acid oxidation, mitochondrial biogenesis and thermogenesis in brown adipose tissue (BAT). CIDEA, cell-death-inducing DNA fragmentation factor A-like effector A; FGF21, fibroblast growth factor 21; PGC1α, PPARγ coactivator 1α; PRDM16, PR domain containing 16; SIRT1, sirutin 1.
Fetal programming of brown adipose tissue by maternal dietary fats
Clearly, UCP-1 gene expression and thermogenesis in BAT are sensible to dietetic changes. Such changes are also closely related to fetal programming( Reference Godfrey 88 , Reference Gabory, Vigé and Ferry 89 ). It is now thoroughly known that not just the amount but also the quality of the maternal diet matters as a variable in the epigenetic regulation in the offspring( Reference Innis 90 ). For this reason, it is important to think about the beneficial effects of nutrition on fetal programming of BAT, setting up thermogenesis and preventing obesity.
Maternal dietary fat composition during gestation and lactation is the most important determinant of the quality of fatty acids that reach the fetus through the placenta, or the infant through breast-feeding( Reference Innis 90 , Reference Novak, Keller and Innis 91 ). Fetuses and newborns can synthesise SFA and MUFA, but they have limited capacity to synthesise long-chain PUFA (LCPUFA)( Reference Larqué, Ruiz-Palacios and Koletzko 92 ). After birth, LCPUFA are transferred to the infant through maternal milk, in which the content of arachidonic acid remains relatively constant, whereas the amount of EPA and DHA are dependent on the maternal dietary habits( Reference Larqué, Ruiz-Palacios and Koletzko 92 ).
Despite the diversity of placental structure between mammal species, placental basic morphology, the main cellular type and function are conserved between many species. Trophoblastic cells make up the placenta and assure a bi-directional flux of appropriate nutrient residues required for the normal growth and maturation of the embryo( Reference Fonseca, Correia-da-silva and Teixeira 93 ). Many maternal dietary cues, such as MUFA and n-3 PUFA( Reference Mennitti, Oliveira and Morais 94 ), foods rich in bioactive compounds( Reference del Bas, Crescenti and Arola-Arnal 95 – Reference Mukai, Sun and Sato 98 ), prebiotics( Reference van Hoffen, Ruiter and Faber 99 ) and some probiotics( Reference Gohir, Ratcliffe and Sloboda 100 ), pass through the placenta and programme different fetal tissues by modulating proteins with the perspective of protecting the organism from many diseases throughout life. Studies on fetal programming and the impact of these nutritional compounds on the BAT are rare.
In humans, BAT development occurs mainly in the third gestational trimester and expands its mass in the early postnatal period, keeping a relatively stable size until adolescence, and gradually regressing with age( Reference Symonds 101 ). A study in mice showed that ageing makes BAT morphology more similar to beige adipose tissue, with unilocular lipid droplets, bigger cellular size and higher TAG content( Reference Sellayah and Sikder 102 ). Such morphologic alterations lead to reduced thermogenic and lipolytic capacity, which may increase the development of diseases( Reference Giralt and Villarroya 103 ).
In 1988, a study revealed that a low-energy diet during pregnancy caused lower birth weight in the rat offspring, and did not change UCP-1 thermogenic activity in BAT. However, when dams that had mild maternal undernutrition during lactation nursed these offspring, they kept lower neonatal body weight, and significantly repressed UCP-1 expression in BAT (assessed on the 4th and 13th day of life). In contrast, when dams were fed a normal energetic diet during lactation, neonatal body weight was restored, and BAT remained unaltered in the offspring. Therefore, it implied that slight maternal undernutrition during lactation could alter UCP-1 expression in offspring BAT( Reference Felipe, Villarroya and Mampel 16 ).
In light of the above, a study using ewes subjected to 30 % energy restriction for 60 d before conception and during gestation caused increased UCP-1 mRNA in perirenal offspring BAT. However, restricted food intake by 50 % fewer energy content in late gestation lowered UCP-1 mRNA and fetal fat depot. Hence, this study showed that different phenotypes could be established depending on the degree of the maternal diet restriction, timing and length of duration( Reference Budge 104 ).
Corroborating, another research finding on ewes treated during late gestation with energy restriction of 40 % of total energy requirements resulted in reduced UCP-1 gene expression, and reduced expression of other genes related to thermogenesis, such as the β3-adrenergic receptor and deiodinase type 2, in the pericardial BAT of newborn offspring. However, these alterations were absent in the 30-d-old offspring( Reference Ojha, Robinson and Yazdani 105 ). On the other hand, early to mid-pregnancy diet restriction followed by ad libitum diet intake increased UCP-1 expression and the expression of genes involved in the browning of adipose tissue, such as bone morphogenetic protein 7 and C/EBPp, in the near-term (140 d) fetus. These alterations were also associated with increased fetal pericardial adiposity and body weight. These results suggested that UCP-1 could re-establish normal levels upon offspring growth, and the rise of this protein associated with pericardial adiposity may be an important factor in neonatal viability( Reference Ojha, Symonds and Budge 106 ).
A study on a high-fat diet (45 % energy from fat) during the postweaning, and preceded by maternal protein undernutrition (9 % of casein) during gestation, did not change the final body weight, energy expenditure and UCP-1 in the interscapular BAT, but increased the adiposity in male mice offspring aged 30 weeks. Though, offspring not exposed to maternal protein restriction (18 % of casein) during pregnancy, only postweaning high-fat diet, increased energy expenditure and UCP-1 mRNA in BAT. The authors concluded that a high-fat diet increases energy expenditure by diet-induced thermogenesis, and this is attenuated in mice when their mothers were fed a protein-restricted diet during gestation and lactation, probably resulting in obesity in adulthood( Reference Sellayah, Dib and Anthony 107 ).
As with total dietary fat quantity, UCP-1 seems to be sensitive to changes in the type of maternal dietary fatty acids in fetal programming. A maternal diet rich in olive oil, butter or margarine from the 14th day of gestation until the 20th day of lactation resulted in increased UCP-1 in the interscapular BAT of 21-d-old male rat offspring from dams fed olive oil, which is rich in oleic acid. In addition, oleic acid was found in the maternal milk and the offspring’s serum, and it correlated positively with lessening weight gain in these animals during lactation( Reference Priego, Sánchez and García 18 ).
Previous studies showed that maternal supplementation with hydrogenated vegetable fats, rich in trans fatty acids, in a normolipidic diet model during pregnancy and lactation led to low-grade inflammation in the subscapular BAT of male Wistar rat offspring after weaning. Furthermore, maternal supplementation with the fruit of juçara palm, rich in MUFA and anthocyanins, studied by this same research group, was associated with repair of the BAT homoeostasis by increased UCP-1 protein expression and protected against stunting and reduced carcass fat deposits( Reference Argentato, Morais and Santamarina 108 ).
Despite the scarcity of studies on this subject, the discussed data suggest mechanisms that are integrating fetal programming in BAT and maternal dietary fatty acids, regulating UCP-1 expression and thermogenesis in the offspring (Fig. 3).
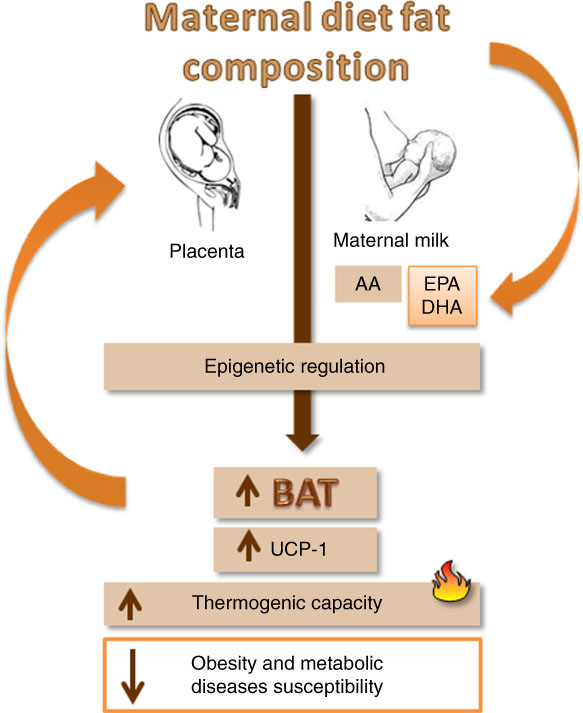
Fig. 3 A schematic diagram showing the impact of maternal nutrition, especially EPA and DHA ingestion, in fetal metabolic programming of brown adipose tissue (BAT) and uncoupling protein 1 (UCP-1) expression and consequently influence on the susceptibility to obesity. AA, arachidonic acid.
Future research directions
The maternal consumption of Western diet in early gestation and during lactation, even in the absence of pre-gestational maternal obesity, can entail increased adiposity, including higher body weight and adipocyte hypertrophy in the offspring after weaning( Reference Bayol, Simbi and Bertrand 109 , Reference Bayol, Simbi and Stickland 110 ). During lactation, maternal diet is critical to the development of obesity and metabolic consequences in the offspring( Reference Bayol, Simbi and Bertrand 109 , Reference Sun, Purcell and Terrillion 111 ). Increased offspring adiposity could be amplified if during lactation the maternal diet is high in fat( Reference Turdi, Ge and Hu 112 ), demonstrating that maternal nutrition is an important regulator of obesity in the offspring. In animal models, there is evidence that maternal dietary fat programme BAT is strong( Reference Felipe, Villarroya and Mampel 16 , Reference Budge 104 – Reference Argentato, Morais and Santamarina 108 ). There is a limitation to human testing for ethical reasons, but the altered UCP-1 expression in offspring BAT from mothers whose diets were high in fat or had a low-quality fat source, for instance hydrogenated vegetable fats, during gestation and lactation could be a possible cause for the increased trend in childhood obesity. Hereby, we remark the importance of more research on the impact of different fatty acids on fetal programming in BAT during different programming periods and in different species. More insights into the epigenetic mechanisms involved in the regulation of UCP-1 expression on fetal programming in future research may contribute to the explanation of various results found in the literature. BAT amount and activity are normally analysed by FDG-PET in combination with CT( Reference Vijgen, Bouvy and Teule 24 ). These techniques require cold condition and expose individuals to ionising radiation, which is not recommended during pregnancy, in newborns and infants until 6 months of age. It is still missing diagnostic tools that allow safe and easy assessment of BAT in humans, in particular during phases of development. With this in mind, future research directions should search for BAT biomarkers that could be easily screened by blood analysis, and that could reflect BAT activity.
Conclusion
The need to understand fetal programming as regards BAT is emphasised by the growing obesity prevalence. Diet is a regulatory factor in the activation of UCP-1 in BAT. A high-fat diet and different fatty acid profiles seem to regulate the UCP-1 expression in BAT positively. However, the molecular mechanisms that explain the activation of UCP-1 in this tissue still need to be more unearthed. There is a limited number of studies looking at the maternal nutrition and BAT development, and it seems that the research trend in this field has been considerably declining since the 1980s. Thus, a better understanding of the impact of a nutrition intervention with fatty acids on the activation of UCP-1 in BAT could lead to new preventive care for metabolic diseases such as obesity.
Acknowledgements
The authors acknowledge the Foundation of São Paulo State Research (FAPSEP) and also the Graduate Program in Food, Nutrition and Health of the Federal University of São Paulo (PPGANS/UNIFESP).
This research was supported by Fundação de Amparo à Pesquisa do Estado de São Paulo (FAPESP) protocol no. 2015/02602-3.
P. P. A. searched and interpreted the literature, wrote the manuscript and drafted the article. H. d. C. C. contributed to writing and editing of the manuscript, and D. E. contributed to the writing and designing of the illustration. L. P. P. conceived and designed the study, revised critically for important intellectual content and approved the final version to be submitted.
The authors declare that they have no conflicts of interest.