The global epidemic rates of obesity, which almost tripled between 1975 and 2016(1), could be attributed to increased intake of unbalanced diet and reduced physical activity(Reference Kopelman2). There are now over 1·9 billion (39 % of the global population) overweight adults in which at least 650 million (13 %) of them are clinically obese. Similarly, about 41 million children under the age of 5 years and 340 million children and adolescents between 5 and 19 years are either overweight or obese(1). Environmental factors such as increased high-energy, low-nutrition food consumption and sedentary lifestyles influence bacterial metabolism including bacteria in the gastrointestinal tract, that is, gut microbiota, which harbours over 1014 bacterial cells from the mouth to the colon(Reference Kobyliak, Virchenko and Falalyeyeva3–Reference Saad, Santos and Prada5).
The number of micro-organisms in the human body is up to ten times higher than that of human cells(Reference Kobyliak, Virchenko and Falalyeyeva3–Reference Saad, Santos and Prada5). Though the microbial composition depends on the organ inhabited, the highest density of microbes is found in the gastrointestinal tract(Reference Kobyliak, Virchenko and Falalyeyeva3–Reference Saad, Santos and Prada5). This ‘microbial organ’ constituted by the microbiota contributes to homoeostasis and influence energy metabolism, insulin sensitivity(Reference Bocci6,Reference Patrice and Nathalie7) and immunological response(Reference Kobyliak, Virchenko and Falalyeyeva3). After an initial decline from the mouth (109) to the stomach (103)(Reference Kobyliak, Virchenko and Falalyeyeva3,Reference Baothman, Zamzami and Taher8) possibly due to increased acidity, the number of micro-organisms increases from the proximal small intestine (about 105) to the colon (about 1012)(Reference Kobyliak, Virchenko and Falalyeyeva3) where there is high density of anaerobes due to the low O2 tension in this region(Reference Villanueva-Millán, Pérez-Matute and Oteo9). Because it contains the highest density of microbes and easily sampled using faeces as a proxy for colonic microbiota, the colon is the most widely studied gut site in relation to microbiota composition and risk of obesity(Reference Kobyliak, Virchenko and Falalyeyeva3). An alteration in the composition of the microbiota within or on the body is associated with many diseases including obesity via several mechanisms. Obesity is fundamentally propagated by a positive energy imbalance, that is, more energy is being consumed than expended(Reference Bocci6,Reference Patrice and Nathalie7) .
The prevalence of obesity continues to increase despite sustained efforts to enlighten the public on the risk of developing chronic adiposity-associated co-morbidities with excessive increase in body weight and obesity. This global epidemic has necessitated the search for better intervention strategies including the exploitation of the health benefits of some gut microbiota and their metabolic products(Reference Borgeraas, Johnson and Skattebu10). In this review, we examined gut microbial composition and the mechanisms of interaction with the host in relation to homoeostasis of energy metabolism and pathophysiology of dysbiosis-induced metabolic inflammation and obesity. We also described the eubiotic, health-promoting influences of probiotics, prebiotics, synbiotics and antibiotics on intestinal microbiota. The role of epigenetic modifications associated with gut microbial dysbiosis and the risk of obesity was also discussed. We searched the literature through PubMed/MEDLINE and Web of Science databases between November 2018 and July 2019 using words and phrases such as (but not limited to) ‘gut microbiota and obesity’, ‘gut microbiome and obesity’, ‘gut microbiota and energy homeostasis or energy metabolism’, ‘obesity-associated gut microbiota’, ‘modulation of gut microbiota’, ‘gut microbiota, obesity and inflammation’. The search included both original research and review articles involving both humans and animal models written in English. Though publication dates were not restricted, articles published within the last two decades and focusing on the gut microbiota-metabolite profiles and inflammatory response in relation to obesity were preferred and included after review/approval by at least two members of the research team. Furthermore, articles discussing only gut microbiota and diabetes mellitus or other diseases (apart from obesity) were excluded.
Microbiota-associated energy harvest
The healthy human gut microbiota consist of over 1000 phylotypes classified into six bacterial divisions/phyla: Firmicutes, Bacteroidetes, Proteobacteria, Fusobacteria, Actinobacteria and Verrucomicrobia(Reference Shen, Obin and Zhao11,Reference Bäckhed, Roswall and Peng12) . While earlier mostly cultivation-dependent studies reported Bifidobacterium and Bacteroides make up 85–98 % of gut microbiota(Reference Hentges13), it is currently believed (using cultivation independent metagenomics technologies) that the gut microbiota comprise mainly (>90 %) Firmicutes and Bacteroidetes(Reference Bäckhed, Roswall and Peng12,Reference Bajzer and Seeley14–Reference Dreyer and Liebl17) (Fig. 1), and sometimes Actinobacteria(Reference Chakraborti18). The changes in gut microbiota associated with obesity are summarised in Table 1(Reference Kobyliak, Virchenko and Falalyeyeva3). The Firmicutes are Gram-positive bacteria and include Lactobacillus, Mycoplasma, Streptococcus and Clostridium, while Bacteroidetes, which are Gram-negative bacteria, include about twenty genera and species, for example, Bacteroides thetaiotaomicron (Reference Bajzer and Seeley14). These organisms are usually benign inhabitants of the intestinal ecosystem coexisting with the host in a commensal and symbiotic relationship. However, a few can be pathogenic especially when they gain access to the peritoneal cavity or systemic circulation(Reference Bajzer and Seeley14).
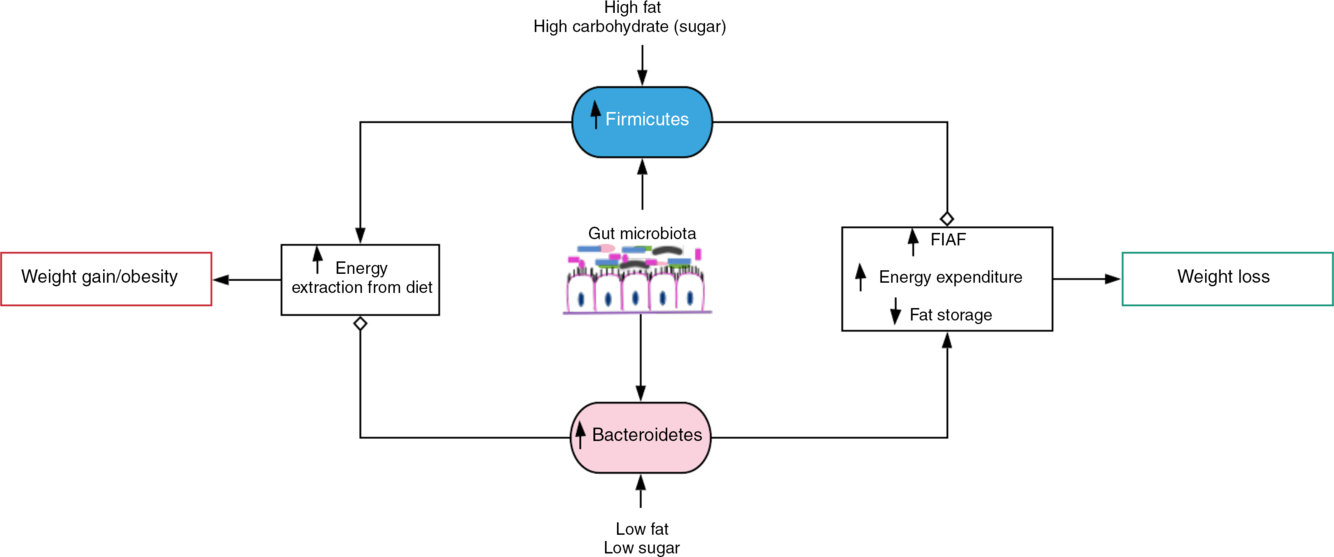
Fig. 1. Gut microbiota-induced energy utilisation. A shift in the gut microbiota in favour of Firmicutes, for example, with consumption of high-fat/carbohydrate diet increases energy extraction from the diet with corresponding weight gain and obesity if left uncontrolled. Contrastingly, consumption of diet low in fat and sugar increases Bacteroidetes dominance, which encourages weight loss by stimulating increased expression of fasting-induced adipocyte factor (FIAF) and subsequent increase in energy expenditure and reduced fat storage. , Stimulation/increase;
, inhibition/decrease.
Table 1. Obesity-associated changes in gut microbiota*

↑, Increase; ↓, decrease.
* Adapted from Kobyliak et al.(Reference Kobyliak, Virchenko and Falalyeyeva3).
Predisposition to increased body fat or obesity is determined by the Firmicutes:Bacteroidetes (F:B) ratio(Reference Dreyer and Liebl17). Obese microbiota exhibit significantly elevated F:B ratio compared with lean gut microbiota with preponderance of Bacteroidetes (up to 50 % more) even when food/energy consumption between the groups is similar. Obese individuals have shown up to 90 % less Bacteroidetes and more Firmicutes than lean individuals(Reference Dreyer and Liebl17,Reference Ley, Bäckhed and Turnbaugh19) . The composition of Bacteroidetes in obese microbiota is increased, while Firmicutes are reduced with corresponding weight loss by decreasing consumption of high-fat/carbohydrate diet(Reference Ley, Bäckhed and Turnbaugh19–Reference Turnbaugh, Ley and Mahowald21). In contrast, with a 209 kJ increase in energy extraction from the diet, a 20 % rise in Firmicutes and a proportionate decrease in Bacteroidetes associated with weight gain have been recorded(Reference Jumpertz, Le and Turnbaugh20). Therefore, an influence of the gut microbiota on host energy harvest, that is, an obesogenic function associated with increased Firmicutes and an antiobesogenic function of Bacteroidetes, was suggested(Reference Ley, Bäckhed and Turnbaugh19,Reference Jumpertz, Le and Turnbaugh20) .
However, these microbiota signatures are not observed in some cases due to confounding factors that affect the composition of the gut microbiota including fasting, composition and energetic content of diet, use of antibiotics(Reference Davis22), age, geographical location(Reference Hu, Park and Jang23), intensity and regularity of exercise(Reference Schwiertz, Taras and Schäfer24), genetic, technical and clinical factors(Reference Hu, Park and Jang23). For instance, an increase in Bacteroidetes over Firmicutes (decreased F:B ratio) was observed in overweight and obese unrestricted human subjects(Reference Schwiertz, Taras and Schäfer24). In other cases, within the same cohort, differences in gut microbiota have been observed at the genus and family levels but not at the phylum level. Recently, a Korean adolescent population showed higher Bacteroides/Bacteroidaceae in the normal-weight group, whereas the obese participants had higher Prevotella/Prevotellaceae. Additionally, the relative abundance of Firmicutes, Bacteroidetes and Proteobacteria; and the F:B ratio did not differ significantly(Reference Hu, Park and Jang23). Furthermore, although there is an overall agreed increase in Firmicutes(Reference Abdallah Ismail, Ragab and Abd Elbaky25), some other studies have attributed the risk of obesity to decrease in the proportion of Actinobacteria (Bifidobacterium)(Reference Murphy, Cotter and Healy26,Reference Turnbaugh, Hamady and Yatsunenko27) or Verrucomicrobia (Akkermansia muciniphila)(Reference Clarke, Murphy and Nilaweera28,Reference Everard, Belzer and Geurts29) and not the F:B ratio (Table 1). Hence, the shift in the gut microbiota in relation to changes in dietary composition could be better interpreted within defined study populations.
The pathogenesis of obesity is partly mediated by gut microbiota(Reference Davis22) (Fig. 1). The gut microbiota is capable of harvesting (metabolising) energy from the diet, for example, metabolising (digesting) the otherwise indigestible dietary fibres. Dietary fibres (polysaccharides and oligosaccharides) as well as proteins, peptides and glycoprotein are converted into products that are readily absorbed by the host such as SCFA – acetate, propionate and butyrate(Reference Chakraborti18). SCFA contribute about 10 % of daily energy requirement and are accountable for almost 75 % of energy metabolism in the colonic epithelium(Reference Chakraborti18,Reference Carvalho and Abdalla Saad30,Reference Rees31) . Therefore, the rate of SCFA metabolism can determine the direction of host energy balance(Reference Chakraborti18).
There are also methanogenic Archaea (Methanobrevibacter smithii) that oxidise (recycle) H2 produced by bacterial species by combining it with carbon dioxide. Fermentation of polysaccharides by bacterial species such as Prevotella is enhanced by increased H2 utilisation by methanogenic Archaea. This H2 transfer between bacterial and archaeal species favours increased energetic uptake by obese individuals(Reference Chakraborti18,Reference Carvalho and Abdalla Saad30,Reference Zhang, DiBaise and Zuccolo32) , although the utility of Archaea as a potential biomarker of obesity has been queried(Reference Schwiertz, Taras and Schäfer24). However, the literature evidence suggests that the gut microbiota determine the differences in the efficiency of energy extraction from diet and energy metabolism in the muscle, liver and adipose tissue(Reference Davis22,Reference Carvalho and Abdalla Saad30) (Fig. 1). Therefore, obesity occurs when there is a positive energy imbalance occasioned by increased energy consumption than expended(Reference Rees31).
Furthermore, metagenomics studies reveal association of obese microbiota (high F:B ratio) with increased starch, galactose and butyrate metabolism due to the high presence of α-amylases and amylomaltases(Reference Turnbaugh, Ley and Mahowald21). There was significantly higher acetate and butyrate production and reduced energy in faecal matter in the obese group compared with their lean counterparts. This indicates a positive relationship between elevated F:B ratio and increased energy harvest from nutrients, lipogenesis and obesity.
Effect of gut microbiota metabolites on host’s energy balance
The gut microbiota interacts with the intestinal epithelial cells through several mechanisms including production of metabolic end products such as SCFA, for example, acetate, butyrate and propionate. As previously stated, these are fermentation products of the degradation of non-digestible carbohydrate and non-carbohydrate substrates in the large intestine. As no bacteria has the capacity to hydrolyse all nutrients and produce all metabolites observed in the gut lumen, there is metabolic synergy among the bacterial community, that is, the entire community collaborate to produce the physiological relationship with the host cells(Reference Carvalho and Abdalla Saad30). Dysregulation of the physiological and biochemical interaction between the host and gut microbiota is characteristic of the obese state(Reference Shen, Obin and Zhao11).
SCFA are absorbed by the intestinal cells by passive diffusion and mono-carboxylic acid transporters, for example, monocarboxylate transporter 1. Apart from being the major energy source for colonic epithelial cells(Reference Rees31), SCFA also perform other metabolic roles. For instance, acetate is a precursor for cholesterol or fatty acid synthesis (lipogenesis), for example, de novo synthesis of lipids in liver(Reference Chakraborti18); propionate is a substrate necessary for gluconeogenesis(Reference Davis22) and reduces food intake and cholesterol synthesis(Reference Chakraborti18); while butyrate is involved in cell growth and differentiation(Reference Carvalho and Abdalla Saad30). Butyrate also protects against diet-induced obesity without causing hypophagia, reduces insulin insensitivity in mice and has obesity-associated anti-inflammatory and anticancer properties in humans, as well as increases leptin gene expression(Reference Chakraborti18).
Acetate and butyrate are also able to promote mitochondrial fatty acid oxidation and energy expenditure via the activation of 5′-AMP-activated protein kinase. 5′-AMP-activated protein kinase phosphorylates and inhibits acetyl-CoA carboxylase, which reduces malonyl-CoA synthesis(Reference Kahn, Alquier and Carling33). Decreased malonyl-CoA activates carnitine:palmitoyl-CoA transferase-1, which enhances uptake and oxidation of long-chain acyl-CoA fatty acids in the mitochondria of liver and muscles leading to weight loss and increase in cholesterol and TAG levels(Reference Saad, Santos and Prada5,Reference Carvalho and Abdalla Saad30,Reference Kahn, Alquier and Carling33) .
Acetate and butyrate also influence intestinal epithelial barrier function by reducing epithelial permeability. They stimulate goblet cells to produce mucin containing mucus, increase the expression of tight junction proteins, for example, zonula occludens-1, zonulin, occludin and claudin, thereby protecting the epithelial cells and decreasing intestinal permeability. This prevents the translocation of intestinal bacteria and lipopolysaccharide (LPS)-induced inflammation(Reference Saad, Santos and Prada5,Reference Carvalho and Abdalla Saad30) .
Furthermore, the SCFA prevent inflammation by inhibiting NF-κB-mediated expression of TNF-α, IL-6, IL-12, etc. (Fig. 2) and increase the expression of IL-10. The SCFA perform this anti-inflammatory action by binding to G protein-coupled receptor 41 and 43 (GPR41 and GPR43) expressed in intestinal epithelial cells. Acetate shows preference for GPR43 through which its anti-inflammatory action is achieved(Reference Carvalho and Abdalla Saad30).
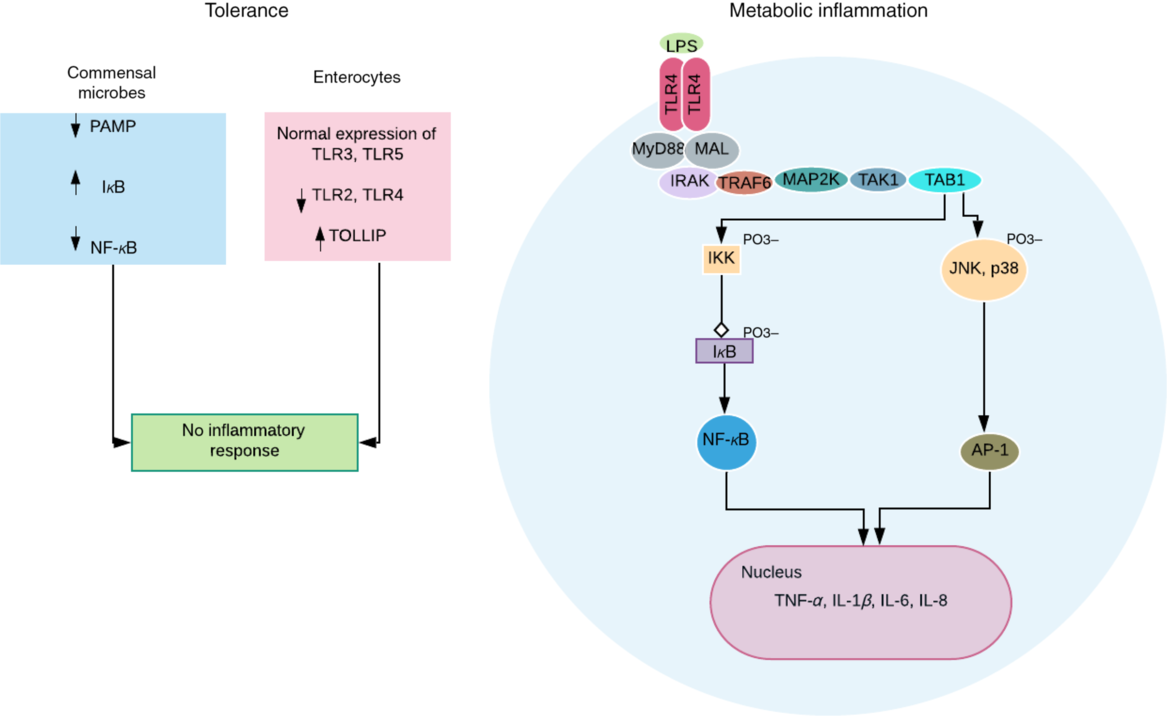
Fig. 2. Obesogenic intestinal host–microbial interaction. In healthy condition, the commensal microbes express reduced levels of pathogen-associated molecular pattern (PAMP) and decreased NF-κB activation due to reduced ubiquitination and proteasomal degradation of IκB (inhibitor of NF-κB). More so, the intestinal epithelial cells express normal levels of Toll-like receptors (TLR) 3 and 5, reduced levels of TLR 2 and 4, and increased levels of TLR-inhibiting peptide (TOLLIP)(Reference Kobyliak, Virchenko and Falalyeyeva3). Both processes ensure a symbiotic relationship and immune tolerance. However, a breach in this relationship, for example, lipopolysaccharide (LPS) translocation can trigger an LPS-induced metabolic inflammation associated with obesity. TLR4 dimerises after binding and activation by LPS. This eventually results in transcription of proinflammatory genes and cytokine secretion by NF-κB (via activation of IKK (IκB kinase) complex) and activator protein-1 (AP-1) (via activation of c-Jun N-terminal kinase (JNK) and mitogen-activated protein kinase (p38)). These intracellular signalling pathways are mediated by adapter molecules – myeloid differentiation primary response protein 88 (MyD88), and MyD88 adapter-like protein (MAL) or Toll-IL-1 receptor adapter protein (TIRAP); and signalling transduction proteins including IL-1R-associated kinase (IRAK); TNF receptor-associated factor-6 (TRAF6); mitogen-activated protein kinase kinase (MAP2K); transforming growth factor β-activated kinase-1 (TAK1); and TAK1-binding protein (TAB1).
The SCFA also partly mediate the expression and activity of anorectic (hunger-suppressing) hormones such as glucagon-like peptide-1 (GLP-1, produced by colonic L-cells), peptide YY (PYY, produced by ileal and colonic cells) and adipose tissue-derived leptin. These hormones act on the hypothalamus to promote satiety and reduce food intake. SCFA are believed to mediate these processes via GPR41(Reference Carvalho and Abdalla Saad30).
Obesogenic metabolic inflammation
Obesity is characterised by a chronic low-grade inflammation propagated by proinflammatory mediators such as TNF-α, IL-1 and IL-6 released by adipocytes. These cytokines stimulate the release of more cytokines and chemokines, and lipogenesis by acting on adipocytes in a paracrine and/or autocrine fashion. The gut epithelium provides the largest body surface for host–microbial interaction promoting tolerance to commensals (Fig. 2), while preventing the growth and proliferation of pathogens via optimal immune responses(Reference Kobyliak, Virchenko and Falalyeyeva3). Gram-negative intestinal bacteria such as Prevotellaceae (high in obese individuals)(Reference Kobyliak, Virchenko and Falalyeyeva3,Reference Chakraborti18) continuously release LPS that along with the bacteria stimulates strong immune response by binding to Toll-like receptor 4 (TLR4) and cluster of differentiation 14 (CD14) receptors on innate immune cells(Reference Cani, Amar and Iglesias34), after translocation into the circulation (Fig. 2). The translocation is aided by increased intestinal permeability due to deficient epithelial barrier associated with decreased SCFA production(Reference Saad, Santos and Prada5). A chronic low-level systemic accumulation of bacteria and LPS results in metabolic bacteraemia (MB)(Reference Amar, Chabo and Waget35) and endotoxaemia (ME)(Reference Cani, Amar and Iglesias34), respectively – proinflammatory processes characteristic of obesity and other metabolic syndrome phenotypes(Reference DiBaise, Frank and Mathur36).
High-fat diet increases adherence of Gram-negative bacteria to the gut mucosa and enhances translocation of the bacteria into circulation and sequestration in the mesenteric lymph due to phagocytosis(Reference Shen, Obin and Zhao11). LPS is also absorbed by enterocytes into the circulation with chylomicrons (fat globules)(Reference Ghoshal, Witta and Zhong37) and transported through the lymphatic fluid to the liver and adipose tissue. ME stimulates obesity and insulin insensitivity(Reference Cani, Amar and Iglesias34). This was observed in normal diet-fed germ-free mice infused with LPS that developed ME symptoms and elevated body fat/weight gain similar to their counterparts on high-fat diet. High-fat diet increases LPS translocation and circulation levels up to 2–3-fold but significantly (10–50 times) lower than septicaemic and infection levels(Reference Cani, Amar and Iglesias34,Reference Amar, Burcelin and Ruidavets38,Reference Brun, Castagliuolo and Leo39) . The LPS-induced metabolic changes are accompanied by reduction of Bacteroides and Bifidobacterium that reduce intestinal LPS levels in mice and improve mucosal barrier function(Reference Griffiths, Duffy and Schanbacher40,Reference Wang, Xiao and Yao41) . Clostridium coccoides belonging to the Firmicutes phyla (Gram positive) was also reduced(Reference Cani, Amar and Iglesias34).
Interestingly, deletion of CD14 delays these obesogenic actions of LPS and high-fat diet(Reference Cani, Amar and Iglesias34), which resumed perhaps due to the interaction of LPS with TLR4(Reference Rees31). NEFA can also bind TLR4 to activate innate immune cells(Reference Shi, Kokoeva and Inouye42) leading to the release of cytokines. However, this high-fat diet-induced effect does not occur in the absence of CD14 and TLR4. Absence of CD14 and TLR4 confers some protection against the metabolic, inflammatory and obesogenic effects of high-fat diet or LPS infusion(Reference Shen, Obin and Zhao11,Reference Cani, Amar and Iglesias34) . Hence, CD14 appears to set the threshold at which metabolic diseases occur(Reference Cani, Amar and Iglesias34). Bacterial translocation and MB are also prevented in high-fat diet-fed mice lacking nucleotide-binding oligomerisation domain-containing protein 1 or CD14(Reference Amar, Chabo and Waget35). Further reading on the host–microbiota interaction, that is, the mechanisms associated with gut epithelial barrier function and integrity, and ME/MB, obesity and insulin resistance can be found in the reviews by Saad et al.(Reference Saad, Santos and Prada5), Shen et al.(Reference Shen, Obin and Zhao11) and Carvalho & Saad(Reference Carvalho and Abdalla Saad30).
Inhibition of angiopoietin-like protein 4
Angiopoietin-like protein 4 also known as fasting-induced adipocyte factor (FIAF) or PPARγ angiopoietin-related protein or hepatic fibrinogen/angiopoietin-related protein is a cell signalling glycoprotein hormone (about 50 kDa) and adipocytokine produced by white adipose tissue, liver, heart, skeletal muscle and intestines(Reference Rees31,Reference Koliwad, Kuo and Shipp43–Reference Yoshida, Shimizugawa and Ono46) . FIAF inhibits lipoprotein lipase activity and stimulates white adipose tissue lipolysis (Fig. 3), with increased circulating levels observed during fasting(Reference Koliwad, Kuo and Shipp43,Reference Mattijssen and Kersten45) .
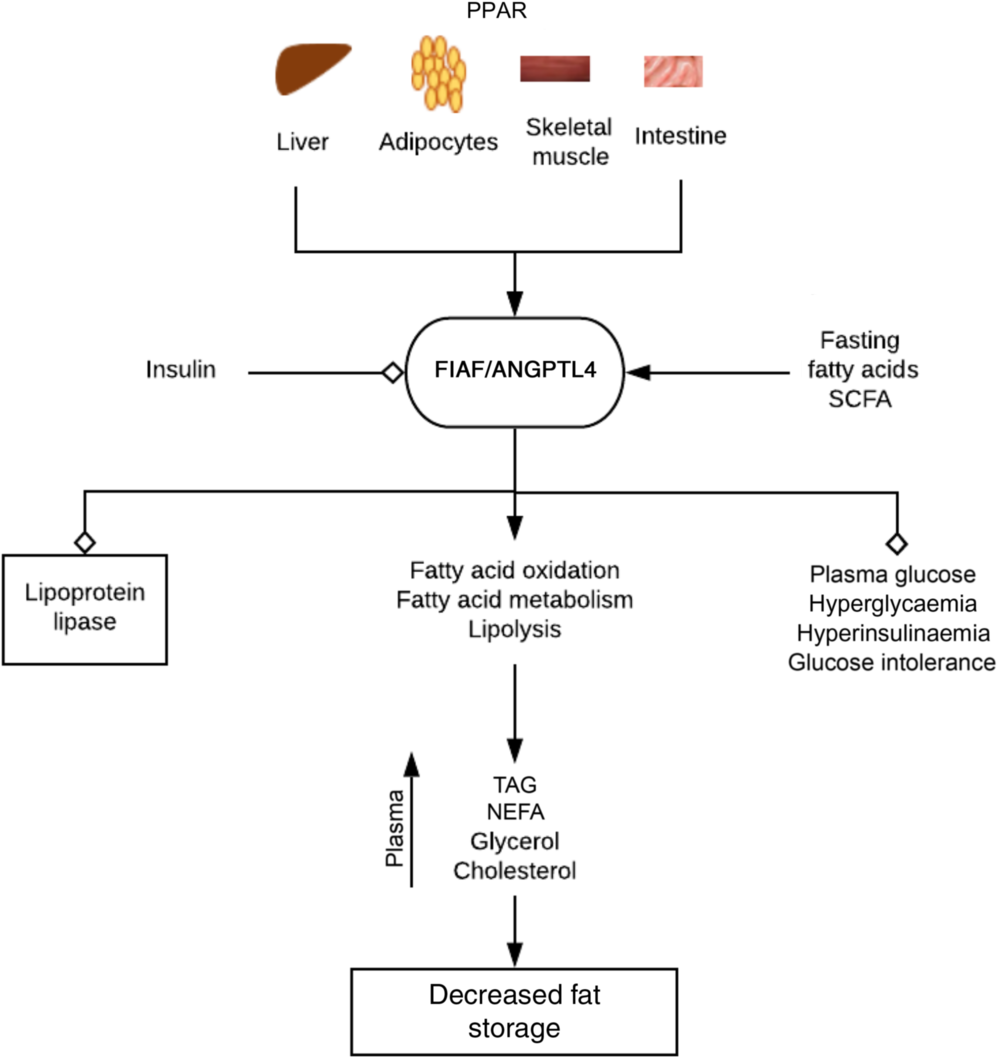
Fig. 3. Regulation of fat metabolism and storage by fasting-induced adipocyte factor (FIAF). By activating PPAR on tissues associated with energy utilisation and fat storage, fasting, fatty acids and bacterial fermentation products such as SCFA increase the expression of FIAF. FIAF is a potent lipoprotein lipase inhibitor and stimulates fatty acid oxidation, metabolism and lipolysis. This results in increased plasma levels of TAG, NEFA, glycerol and cholesterol culminating in depletion of fat storage and hence decreased body weight and obesity. FIAF also decreases plasma glucose levels, hyperinsulinaemia and glucose intolerance, while insulin inhibits its expression. ANGPTL4, angiopoietin-like protein 4. , Stimulation/increase;
, inhibition/decrease.
Lipoprotein lipase hydrolyses lipoprotein-associated TAG to NEFA, which are re-esterified into TAG and stored as fat in peripheral white adipose tissue (lipogenesis). FIAF stimulates fatty acid oxidation and fat mobilisation leading to a reduction in adipose tissue mass. Increased FIAF expression increases plasma levels of TAG, NEFA, glycerol, HDL-cholesterol and total cholesterol(Reference Mandard, Zandbergen and van Straten44). FIAF may also decrease plasma glucose level, return hyperglycaemia to normal level and attenuate hyperinsulinaemia and glucose intolerance(Reference Xu, Lam and Chan47). Fatty acids stimulate expression of FIAF in mice and humans (especially in fasting, prolong energetic restriction and exercise) by activating PPARα (liver, small intestine), γ (adipose tissue, colon) and δ (heart, skeletal muscle, macrophages)(Reference Mattijssen and Kersten45), whereas insulin suppresses FIAF expression(Reference van Raalte, Brands and Serlie48) (Fig. 3). The contrasting action of fasting and insulin on FIAF regulation may account for the shift in energy utilisation from fatty acids derived from lipoprotein after a meal to NEFA during fasting(Reference Mattijssen and Kersten45). The glucocorticoids-induced flux of TAG from white adipose tissue to the liver is also mediated by FIAF(Reference Koliwad, Kuo and Shipp43).
Decreased FIAF expression has been observed in germ-free mice colonised by normal caecal microbiota with a corresponding increase in adipocyte lipoprotein function and total fat mass content by 122 and 57 %, respectively(Reference Bäckhed, Ding and Wang49). Though the exact micro-organisms were not identified, stimulation of lipogenesis (deposition of TAG in adipocytes) via microbiota-induced suppression of FIAF expression was implicated(Reference Bäckhed, Ding and Wang49). Bacterial fermentation products such as SCFA have also shown ability to promote increased FIAF expression through PPARγ in colon cells(Reference Carvalho and Abdalla Saad30). FIAF is a potent regulator of lipid metabolism and adiposity, dysregulation of which might result in dyslipidaemia(Reference Mandard, Zandbergen and van Straten44). Further reading on the physiological role of FIAF and other angiopoetin-like proteins on lipid/NEFA metabolism can be found in Mattijssen & Kersten(Reference Mattijssen and Kersten45).
Modulation of gut microbiota
The global burden of obesity has triggered the search for suitable bespoke intervention strategies including the exploration of the eubiosis and health-promoting effects of some gut microbiota and their metabolic products(Reference Borgeraas, Johnson and Skattebu10). A food design strategy modifying the microbiota combined with altered food intake rather than medical(Reference Carvalho and Abdalla Saad30,Reference Aronsson, Huang and Parini50) or surgery-based(Reference Carvalho and Abdalla Saad30,Reference Seganfredo, Blume and Moehlecke51) interventions appears more cost-effective and readily accessible by a larger population.
Probiotics
As a consequence of the relationship between gut microbiota and obesity, modulation of the microbiota by probiotics could promote weight loss, reduce BMI and fat percentage(Reference Borgeraas, Johnson and Skattebu10), hence, be employed in treatment of obesity or as a prophylactic treatment for those at risk. Probiotics are viable micro-organisms that have health-promoting effects on the host when administered in adequate amounts as food ingredients(Reference Shen, Obin and Zhao11,Reference Davis22,Reference Rastall, Gibson and Gill52) . The effect of Lactobacillus, Bifidobacterium and Saccharomyces on weight loss and/or fat deposition in overweight adults has been reported(Reference Rastall, Gibson and Gill52,Reference Crovesy, Ostrowski and Ferreira53) . More than 60 % of the studies demonstrated Lactobacillus species-dependent decrease in body weight and/or body fat. For example, weight loss is enhanced by Lactobacillus gasseri and Lactobacillus amylovorus; hypoenergetic diet combined with Lactobacillus plantarum and Lactobacillus rhamnosus; L. plantarum combined with Lactobacillus curvatus; and combining Lactobacillus acidophilus, Lactobacillus casei and phenolic compounds.
Forty-three overweight humans who consumed 200 g of fermented milk containing L. gasseri per d for 12 weeks experienced significant reduction in abdominal visceral and subcutaneous fat, body weight, BMI and waist and hip circumference compared with those that had unsupplemented fermented milk(Reference Kadooka, Sato and Imaizumi54). Supplementation of high-fat diet with Lactobacillus paracasei F19 and Bifidobacterium lactis, but not B. thetaiotaomicron, up-regulates FIAF expression and concomitant anti-obesogenic benefit to the host(Reference Rees31,Reference Aronsson, Huang and Parini50) , for example, reducing serum TAG levels and alleviating fat deposition in liver(Reference Yin, Yu and Fu55). Similarly, mice supplementation with Bifidobacterium infantis and Bifidobacterium bifidum for about a month showed significantly reduced gut endotoxin levels with no increase in IL-6, TNF-α, INF-γ (Reference Griffiths, Duffy and Schanbacher40). Treatment of high-fat diet-fed mice with Bifidobacterium animalis subsp. Lactis 420 improves overall inflammatory and metabolic status by reversing/preventing bacterial translocation through the intestinal epithelium(Reference Amar, Chabo and Waget35). Additionally, treatment with mucin-degrading A. muciniphila was associated with improved metabolic profile achieved by reversing fat deposition, ME, adipose tissue inflammation and insulin resistance induced by high-fat diet. Treatment with A. muciniphila or its extracellular vesicles also increases intestinal levels of endocannabinoids that enhance gut epithelial barrier function by increasing expression of occludin and decreasing intestinal permeability, as well as controls inflammation and gut peptide secretion(Reference Everard, Belzer and Geurts29,Reference Chelakkot, Choi and Kim56) .
Yeast probiotics such as Saccharomyces boulardii also improve the microbiota-metabolic profiles of genetically obese and diabetic mice to one with reduced Firmicutes and increased Bacteroidetes with a corresponding decrease in fat mass and circulating inflammatory mediators(Reference Osterberg, Boutagy and McMillan57). In addition, multispecies probiotics such as VSL#3 also influence (restore) gut microbiota profile and promote epithelial tight junction integrity and anti-inflammation(Reference Carvalho and Abdalla Saad30,Reference Chibbar, Alahmadi, Dieleman, Floch, Ringel and Allan Walker58) , thereby preventing fat accumulation and weight gain(Reference Davis22,Reference Everard, Belzer and Geurts29) . VSL#3 is a commercial probiotic mixture of lactobacilli (L. casei, L. plantarum, L. acidophilus and Lactobacillus delbrueckii subsp. bulgaricus); bifidobacteria (Bifidobacterium longum, Bifidobacterium breve and B. infantis) and Streptococcus (Streptococcus salivarius subsp. thermophilus)(Reference Shen, Obin and Zhao11,Reference Chibbar, Alahmadi, Dieleman, Floch, Ringel and Allan Walker58) .
In contrast to the health-promoting (antiobesogenic) effects of probiotics, some studies have reported no health benefit or even obesogenic effects associated with probiotics. For instance, enhanced weight gain observed in livestock that were fed huge amounts of probiotics has stimulated speculations that the obesity pandemic in humans may be associated with high consumption of foods enriched with probiotic bacteria(Reference Raoult59). Two meta-analyses also suggested that probiotics may promote weight gain in children and undernourished individuals while promoting weight loss in adults and obese individuals(Reference Dror, Dickstein and Dubourg60,Reference Million, Angelakis and Paul61) . These discrepancies have been attributed to differences in probiotic bacterial strain administered as well as age and baseline body weight of the host(Reference Davis22). Therefore, more comprehensive randomised controlled trials are required to ascertain the efficacy of probiotics in the management of obesity locally or globally.
Prebiotics
Prebiotics are non-digestible food ingredients (usually polysaccharides) capable of selectively stimulating growth and/or activity of microbiota especially lactobacilli and bifidobacteria, thereby providing health-promoting effects on host energy balance(Reference Roberfroid, Gibson and Hoyles62,Reference Lim, Ferguson and Tannock63) . They modify the microbiota to mitigate the risk of dysbiosis and associated gut and systemic pathologies, that is, they restore and/or maintain eubiosis or normobiosis(Reference Roberfroid, Gibson and Hoyles62).
Most fermentable dietary fibres particularly non-digestible oligosaccharides such as fructo-oligosaccharides (e.g. inulin), galacto-oligosaccharides and resistant starch(Reference Lim, Ferguson and Tannock63) that occur in several foods(Reference Kolida and Gibson64) have shown capacity to alter gut microbiota. They also increase satiation by up-regulating GLP-1 and PYY and decrease secretion of ghrelin, thereby reducing food intake(Reference Shen, Obin and Zhao11,Reference Carvalho and Abdalla Saad30,Reference Delzenne, Cani and Daubioul65,Reference Cani, Possemiers and Van de Wiele66) . An example is the weight loss, decreased ghrelin expression, increase in PYY levels, low energy intake, low plasma glucose and insulin levels experienced by forty-eight adults with BMI > 25 kg/m2 who received 21 g/d of oligofructose for 12 weeks(Reference Patrice and Nathalie7). Similarly, obese pre-menopausal women who ingested oligofructose-rich syrup (0·14 g fructo-oligosaccharides/kg per d) for 120 d experienced a dramatic weight loss (up to 15 kg) during the study accompanied by decreased fasting insulin and LDL-cholesterol levels(Reference Genta, Cabrera and Habib67).
Prebiotics are fermented to metabolically active SCFA by bacteria in the large intestine. It is believed that the antiobesogenic effects of prebiotics are mediated, at least partly, by the SCFA including acetate, propionate and butyrate(Reference Shen, Obin and Zhao11,Reference Carvalho and Abdalla Saad30) . They also enhance gut mucosal integrity and barrier function by increasing Bifidobacterium population(Reference Cani, Possemiers and Van de Wiele66,Reference Cani, Neyrinck and Fava68) , which is known to produce butyrate(Reference Seganfredo, Blume and Moehlecke51).
Synbiotics and antibiotics
For a more beneficial effect, a combination of probiotics and prebiotics, which is termed synbiotics, has been employed. For example, combining oligofructose-enriched inulin, Lactobacillus rhammnosus and B. lactis, altered the microbiota in favour of Lactobacillus and Bifidobacterium at the expense of Clostridium perfringens (Reference Rafter, Bennett and Caderni69). This appears to be more efficacious than either probiotics or prebiotics singly and has been demonstrated in in vitro studies(Reference Saulnier, Gibson and Kolida70) but requires further investigation in humans(Reference Beserra, Fernandes and do Rosario71).
Additionally, broad-spectrum antibiotic therapy has been employed in mice to reduce intestinal permeability by increasing tight junction protein expression, reduce LPS-induced inflammation and improve obesity-induced insulin resistance in the liver, muscle and adipose tissue. These effects are plausibly achieved through the reduction of circulating levels of LPS and TLR4 activation. Portal acetate levels, which activate 5′-AMP-activated protein kinase, fatty acid oxidation and energy expenditure (Fig. 3), also increase with antibiotic treatment. However, antibiotic resistance, ability to alter commensal bacterial community and the possible association of chronic low-dose antibiotic use and weight gain have hindered successful translation to humans(Reference Carvalho and Abdalla Saad30).
The mechanisms underpinning this probiotic-induced modulation of gut microbiota promoting weight loss and reduced fat mass include decreasing gut permeability by maintaining epithelial cell tight junctions, preventing translocation of whole bacteria, their products and metabolites thereby decreasing LPS-induced inflammation. The reduced inflammation enhances leptin and insulin sensitivity in the hypothalamus that improves satiety and glucose tolerance. Improved hypothalamic insulin sensitivity combined with increased concentrations of GLP-1 and PYY leads to increased satiety and reduced food intake. Reduced energy consumption and elevated FIAF expression encourage weight loss and reduced fat deposition(Reference Carvalho and Abdalla Saad30).
Bariatric surgery has also been shown to increase gut microbial diversity by reducing the relative abundance of Firmicutes and increasing the abundance of Bacteroidetes, that is, decreased F:B ratio. This was reported in a recent systematic review that included studies employing the two main variants of bariatric surgery, that is, Roux-en-Y gastric bypass and the laparoscopic sleeve gastrectomy(Reference Castaner, Goday and Park72).
Diet-microbiota-dependent epigenetic changes
An epigenetic trait is a ‘stably heritable phenotype resulting from changes in a chromosome without alterations in the DNA sequence’(Reference Berger, Kouzarides and Shiekhattar73). Hence, epigenetics could be defined as the study of heritable phenotype alterations that occur without changes in DNA sequence(Reference Dupont, Armant and Brenner74). These changes are triggered by factors including diet, ageing, drugs, environmental chemicals, etc.(Reference Berger, Kouzarides and Shiekhattar73). The possibility of epigenetic alterations underlying certain host responses to changes in the gut microbiome has been highlighted. Dysbiosis alters both the transcriptome and proteome of intestinal epithelium(Reference Camp, Frank and Lickwar75–Reference Qin, Roberts and Grimm77). Gut microbiota regulates gene expression through DNA methylation in intestinal epithelium independent of DNA methyltransferase. The DNA methylation changes can be reproduced by faecal transplantation(Reference Yu, Gadkari and Zhou78). Chromatin structure in intestinal intraepithelial lymphocytes(Reference Semenkovich, Planer and Ahern79) and several host tissues is also modified by gut microbiota in a diet-dependent manner(Reference Krautkramer, Kreznar and Romano80). The result is alterations in gene transcription/expression and host physiology(Reference Krautkramer, Kreznar and Romano80).
Obesogenic diet programmes the gut microbiota to one deficient in bifidobacteria (that elaborates SCFA particularly butyrate, improves tight junction protein expression, maintains epithelial barrier integrity and reduces proinflammatory cytokine expression in mucus)(Reference Ewaschuk, Diaz and Meddings81). Reduced amounts of the beneficial micro-organisms also encourage ME and MB(Reference Shen, Obin and Zhao11,Reference Cani, Amar and Iglesias34,Reference Amar, Chabo and Waget35) . A low-grade chronic inflammation ensues which is a hallmark of obesity and related diseases such as diabetes(Reference Saad, Santos and Prada5,Reference Amar, Chabo and Waget35,Reference Amar, Serino and Lange82) and cancer(Reference Qin, Roberts and Grimm77,Reference Hanahan and Weinberg Robert83) . Both high-fat diet and a dysbiotic gut microbiota are required to epigenetically programme the host for obesity, diabetes and colorectal cancer. This is mediated by the bacterial metabolites produced from the host diet that are capable of altering gene expression(Reference Saad, Santos and Prada5,Reference Qin, Roberts and Grimm77,Reference Li, Grimm and Chrysovergis84) .
Microbiota metabolite-induced alteration in gene expression was observed to be regulated by hepatocyte nuclear factor 4α (HNF4α), a nuclear receptor capable of activating or repressing gene transcription(Reference Qin, Roberts and Grimm77). It is plausible that fatty acids produced from bacterial metabolism of high-fat diet bind to HNF4α because lipids have been identified as HNF4α-binding ligands(Reference Chandra, Huang and Potluri85). Obesity is associated with down-regulation of genes enriched in HNF4α binding sites with slightly higher levels of HNF4α at such sites in obese mice. Animals that received obesogenic diet and microbiota showed down-regulation of genes gaining HNF4α binding(Reference Qin, Roberts and Grimm77).
Mother-to-child transmission of obesity
An offspring could be a product of the combination of the dietary patterns of both the offspring and mother. Prenatal exposure to adverse (obesogenic) microbiota-metabolic environment may increase the offspring’s susceptibility to obesity in postnatal life(Reference Semenkovich, Planer and Ahern79). This has been demonstrated by examining maternal mechanisms regulating developmental programming of offspring obesity in rats(Reference Rees31,Reference Paul, Bomhof and Vogel86,Reference Paul, Collins and Bomhof87) . Maternal obese microbiomial-metabolite phenotype can programme the offspring’s risk of obesity. The ability of prebiotics to encourage the growth and metabolism of distinct health-promoting gut microbiota was explored to mitigate the intergenerational transmission of obesity to offspring(Reference Paul, Bomhof and Vogel86).
Pregnant Sprague–Dawley rats became obese after 2 weeks of feeding on high-fat/sucrose diet. This diet-induced obesity was associated with lower relative abundance of faecal Bifidobacterium sp. and Lactobacillus and higher Clostridium and Methanobrevibacter sp.(Reference Paul, Bomhof and Vogel86,Reference Paul, Collins and Bomhof87) . These changes are consistent with the increased energy harvest and obesity associated with high F:B ratio, and the contrasting improved intestinal epithelial barrier integrity promoted by Bifidobacterium supplementation(Reference Rees31). Diet-induced obese dams also showed higher blood glucose, plasma insulin, fasting plasma leptin (produced in proportion to fat mass) and lower PYY. Furthermore, they showed increased levels of ketone bodies and metabolites involved in lipid metabolism. Elevated branched chain amino acids (associated with increased insulin resistance) and decreased glucogenic amino acids were also identified in the obese dams(Reference Paul, Bomhof and Vogel86).
Expectedly, supplementing the maternal high-fat/sucrose diet with oligofructose prebiotic increased the relative abundance of Bifidobacterium sp. and Bacteroides sp. and reduced Clostridium and Methanobrevibacter sp. There were also increased plasma levels of PYY, GLP-1 and GLP-2. Their serum metabolite profiles were characterised by elevated levels of gut microbial metabolites (propionate, acetate, butyrate, isobutyrate, formate, etc.) and markers of increased insulin sensitivity (myo-inositol). Amino acids involved in arginine metabolism that are associated with improved pregnancy outcomes and reduced offspring adiposity were also identified. Consequently, there was a reduction in energy intake and maternal gestational weight gain. Therefore, maternal prebiotic supplementation in pregnancy and lactation encourages the preponderance of antiobesogenic microbiota associated with reduced fat mass, weight loss and improved insulin sensitivity(Reference Paul, Bomhof and Vogel86).
In the offspring, maternal oligofructose supplementation was associated with lower fat mass, percentage body fat and fasting plasma glucose but similar insulin concentrations compared with offspring from non-supplemented dams. Maternal adiposity correlated with offspring adiposity. Maternally supplemented offspring also displayed higher levels of satiety hormones – PYY, GLP-1 and GLP-2. These metabolic and hormonal/behavioural changes suggest decreased energy intake, improved glycaemic control and reduced risk of insulin resistance and diabetes in postnatal life of offspring born to prebiotic-supplemented dams(Reference Paul, Bomhof and Vogel86). The reduced adiposity observed in offspring of prebiotic-supplemented dams was attributed to the distinct maternal metabolite signature. Similarly, children born to mothers who consumed a probiotic strain (L. rhamnosus GG) continuously from 4 weeks before delivery to 6 months after delivery were prevented from excessive weight gain in their first decade of life(Reference Luoto, Kalliomäki and Laitinen88). Apart from improving our understanding of the maternal mechanisms associated with the developmental programming of offspring obesity, these observations highlight a potential strategy to improve maternal and offspring metabolic adaptive outcomes in pregnancy(Reference Paul, Bomhof and Vogel86,Reference Paul, Collins and Bomhof87) .
Conclusion
High-fat/carbohydrate diet programmes the gut microbiota to one deficient in Bacteroides, Bifidobacterium, Lactobacillus and Akkermansia and rich in Firmicutes (Clostridium), Prevotella and Methanobrevibacter. These alterations in bacterial species composition are associated with decreased expression of SCFA that improve tight junction protein expression, maintain intestinal epithelial barrier integrity, reduce bacterial translocation and proinflammatory cytokine expression(Reference Ewaschuk, Diaz and Meddings81) and increase expression of hunger-suppressing hormones. Reduced amounts of the beneficial micro-organisms also encourage ME and MB(Reference Shen, Obin and Zhao11,Reference Cani, Amar and Iglesias34,Reference Amar, Chabo and Waget35) and reduced FIAF expression leading to dyslipidaemia. A low-grade chronic inflammation ensues that culminate in obesity and associated diseases such as diabetes(Reference Saad, Santos and Prada5,Reference Amar, Chabo and Waget35,Reference Amar, Serino and Lange82) and cancer(Reference Qin, Roberts and Grimm77,Reference Hanahan and Weinberg Robert83) . The synergy of high-fat diet and dysbiotic gut microbiota creates a recipe that epigenetically programmes the host for increased adiposity and poor glycaemic control. Interestingly, these obesogenic mechanistic pathways that are sometimes transmitted from one generation to another can be modulated through the administration of probiotics, prebiotics and synbiotics. Though the influence of gut microbiota on the risk of obesity and several intervention strategies have been extensively demonstrated in animal models, application in humans still requires further robust investigation.
Acknowledgements
This research received no specific grant from any funding agency, commercial or not-for-profit sectors.
The review idea was conceptualised by E. A. and F. O. R. E. A., T. A., E. S. F. O. and F. O. R. contributed to the literature search, drafting and critical revision of the manuscript with E. A. leading the process. All authors read and approved the final draft before submission for publication.
The authors declare that there are no conflicts of interest.