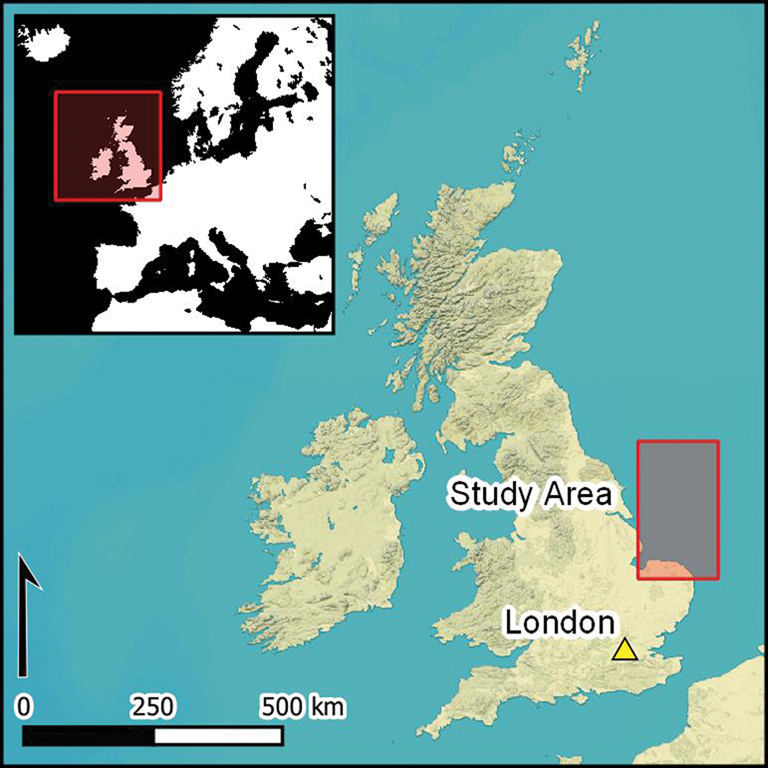
Introduction
In an age of human-induced climate change, catastrophic natural disasters appear to be occurring with greater frequency and magnitude. Tsunamis, such as the 2004 Indian Ocean ‘Boxing Day’ and 2011 Tōhoku (Japan) events, are of particular note, striking quickly and with little warning (Seneviratne et al. Reference Seneviratne and Field2012). Although such events have fuelled interest in how people in the past responded to natural disasters (e.g. Burroughs Reference Burroughs2005; Cain et al. Reference Cain, Goff and McFadgen2018), archaeology has—with few exceptions (e.g. McFadgen Reference McFadgen2007)—been slow to engage in the debate beyond historically attested examples, leaving the sciences to take the lead in palaeotsunami research (Goff et al. Reference Goff, Chagué-Goff, Nichol, Jaffe and Dominey-Howes2012; Engel et al. Reference Engel, Pilarczyk, May, Brill and Garrett2020).
The Storegga tsunami (c. 8150 cal BP) provides a comparative phenomenon within North-west European prehistory. It is geologically well attested (Figure 1), with evidence from Western Scandinavia, the Faroe Isles, north-east Britain, Denmark and Greenland. Caused by the largest known Holocene submarine landslides (80 000km2), the event displaced 3200km3 of sediment (Haflidason et al. Reference Haflidason, Lien, Sjerup, Forsberg and Bryn2005) on the European continental shelf west of southern Norway. The resulting tsunami must have been of considerable proportions (Bugge Reference Bugge1983; Bugge et al. Reference Bugge, Befring, Belderson, Eidvin, Jansen, Kenyon, Holtedahl and Sejrup1987; Dawson et al. Reference Dawson, Long and Smith1988; Long et al. Reference Long, Smith and Dawson1989a), and has even been posited as causing the final inundation of Mesolithic Doggerland, the now submerged palaeolandscape of the southern-central North Sea (Weninger et al. Reference Weninger2008). Specifically archaeological evidence for the tsunami, however, is scarce.
To date, only two Mesolithic sites have been confirmed as underlying Storegga deposits (Figure 1; Bondevik Reference Bondevik and Meyers2019: 29), although others remain a possibility (e.g. Bondevik Reference Bondevik2003). Hijma (Reference Hijma2009: 140–41) notes that “an important question is whether the Storegga tsunami had sufficient force to create a distinct deposit where it dissipated on the southern North Sea shores” or whether “most of the energy had already dissipated […] across the shallowest parts of the contemporary North Sea” (see also Cohen & Hijma Reference Cohen and Hijma2008). The dearth of archaeological evidence suggests the need to examine why archaeologists struggle to define natural disasters in the past. In doing so, this article reviews models of the Storegga tsunami's impact. Using new data (Gaffney et al. Reference Gaffney2020), we re-evaluate the fate of Doggerland and consider the first offshore evidence for the tsunami from the southern-central North Sea.
The archaeology of natural disasters
The archaeology of natural disasters is an emerging field (Faas & Barrios Reference Faas and Barrios2015) that provides the capacity to assess such events in terms of longer-term impacts on lifeways, whereas studies of contemporary natural disasters tend to concentrate on the immediate loss of life (Torrence & Grattan Reference Torrence, Grattan, Torrence and Grattan2002). The devastation wrought by the Indian Ocean and Tōhoku tsunamis, for example, led to both being labelled as ‘mega-tsunamis’ in the media, although neither meet the technical criteria for this description (Goff et al. Reference Goff, Terry, Chagué-Goff and Goto2014: 13). Clearly, scales of time, space and consequence are important for how we understand catastrophic phenomena (see Estévez Reference Estévez, Buchet, Rigeade, Séguy and Signoli2008). It may seem obvious that for a natural disaster to qualify as truly catastrophic, a society must have struggled or failed to adapt to changes in their environment (Oliver-Smith Reference Oliver-Smith1996: 303). Intuitively, however, we recognise that ‘catastrophes’ and ‘natural disasters’ may be understood and experienced with a high degree of subjectivity.
Prehistoric catastrophes and the marine environment
Underwater survey and excavation techniques are among the fastest developing areas of methodological advancement in archaeology (see Fitch et al. Reference Fitch, Thomson and Gaffney2005; Bailey et al. Reference Bailey, Harff and Sakellariou2017; Sturt et al. Reference Sturt, Flemming, Carabias, Jöns and Adams2018). Despite this, data collection beyond the near-shore remains challenging. The difficulties of identifying specific events in such environments—even at the scale of the Storegga slide—remain significant. Consequently, the Storegga tsunami exemplifies the paradoxical scenario described by Torrence and Grattan (Reference Torrence, Grattan, Torrence and Grattan2002: 4) whereby archaeological evidence for the impact of an unusually destructive force upon extant cultures remains elusive. There is an unwitting tendency to adopt a progressivist lens and to presume that hunter-gatherers and early farmers of the past and present were and are less capable of dealing with extreme environmental forces (Bettinger et al. Reference Bettinger, Garvey and Tushingham2015: 12). This is compounded by challenges in identifying catastrophic events archaeologically on conventional, terrestrial sites—particularly in prehistory, where hiatuses in deposition may result from many processes. The transient nature of even ‘permanent’ hunter-gatherer settlements raises questions about how we might recognise responses to environmental change (Moe Astrup Reference Moe Astrup2018: 136).
In many cases, the effects of catastrophic natural disasters may be easier to recognise than evidence for the disaster itself. Goff et al. (Reference Goff, Chagué-Goff, Nichol, Jaffe and Dominey-Howes2012) offer guidelines for identifying archaeological proxies of palaeotsunamis. These include changes in shell-midden composition, structural damage, geomorphological changes (e.g. uplift, subsidence and/or compaction) and replication of these features across multiple sites. In European Mesolithic contexts, however, these proxies can be elusive, as, for example, structural evidence is rare, and variation in shell-midden composition may be attributed to multiple causes.
Searching for Storegga
After initial reports of the geological indicators of the Storegga tsunami emerged in the 1980s, evidence from archaeological deposits soon followed (Dawson et al. Reference Dawson, Smith and Long1990). Since then, however, the number of associated archaeological sites has remained small (Figure 1; Bondevik Reference Bondevik2003). This may reflect a lack of access to appropriate geological expertise (cf. Long et al. Reference Long, Dawson and Smith1989b: 535), difficulties in distinguishing tsunami deposits from storm surges and other transgressive episodes (Bondevik et al. Reference Bondevik, Svendsen and Mangerud1998), or a lack of contemporaneous archaeological sites. Alternatively, the evidence may simply not exist: a tsunami needs a run-up of only 1m to be catastrophic, yet less than 5m is often too little to leave a geological record (Lowe & de Lange Reference Lowe and de Lange2000: 403). Furthermore, tsunamis may erode the landscape, removing any archaeological evidence, while remaining cultural debris may have been cleared by returning people, or simply missed through limited excavation strategies. Hence, a tsunami may cause terrible damage, but leave little archaeological evidence. Finally, the Storegga tsunami coincided with the harshest conditions of the 8.2 ka ‘cold snap’ (Dawson et al. Reference Dawson, Bondevik and Teller2011; Bondevik et al. Reference Bondevik, Stormo and Skjerdal2012; Rydgren & Bondevik Reference Rydgren and Bondevik2015). Separating tsunami impacts from those resulting from this broader climatic downturn is challenging.
The proxies outlined by Goff et al. (Reference Goff, Chagué-Goff, Nichol, Jaffe and Dominey-Howes2012) have so far been lacking from the North Sea basin, with archaeological evidence comprising either unconfirmed tsunami deposits or speculative stratigraphic relationships. Even where Storegga deposits are confirmed as overlying Mesolithic occupations—including Castle Street in Scotland, and Dysvikja, and probably Fjørtoft, in Norway (Dawson et al. Reference Dawson, Smith and Long1990; Bondevik Reference Bondevik2003, Reference Bondevik and Meyers2019)—these cannot be taken as reliable evidence of immediate event impact (Bondevik Reference Bondevik and Meyers2019: 29; Table S1 in the online supplementary material (OSM)).
Reconstructions of what happened to Doggerland rely on terrestrial, primarily geological, data, and have been impeded by a lack of clarity as to what constitutes a contemporaneous landmass. Some have envisioned that Doggerland had all but disappeared prior to Storegga (e.g. Edwards Reference Edwards and Saville2004: 67), while others have proposed that the tsunami constituted the final inundation process (Weninger et al. Reference Weninger2008: 13). Others still have suggested that at the time of the tsunami, Doggerland had already become a (populated) island (Hill et al. Reference Hill, Avdis, Mouradian, Collins and Piggott2017: 1). Assessing these different interpretations requires a nuanced history of the southern North Sea landscape.
A three-stage history of gradual inundation
The integration of palaeobathymetry and seismic analyses (e.g. Gaffney et al. Reference Gaffney, Thomson and Fitch2007), and progress in refining estimates of sea-level rise (Hijma et al. Reference Hijma and Cohen2010, Reference Hijma and Cohen2019; Shennan et al. Reference Shennan, Bradley and Edwards2018; Emery et al. Reference Emery, Hodgson, Barlow, Carrivick, Cotterill, Mellett and Booth2019), have allowed us to characterise the nature and extent of the prehistoric landmass in the southern North Sea (Figure 2). ‘Doggerland’ typically refers to the entire submerged landscape stretching from the Dover/Calais Strait to the Norwegian Sea (Coles Reference Coles1998). This landmass, however, would have changed significantly in extent and character since the Last Glacial Maximum, and the ‘catch-all’ name of Doggerland may not be particularly useful.
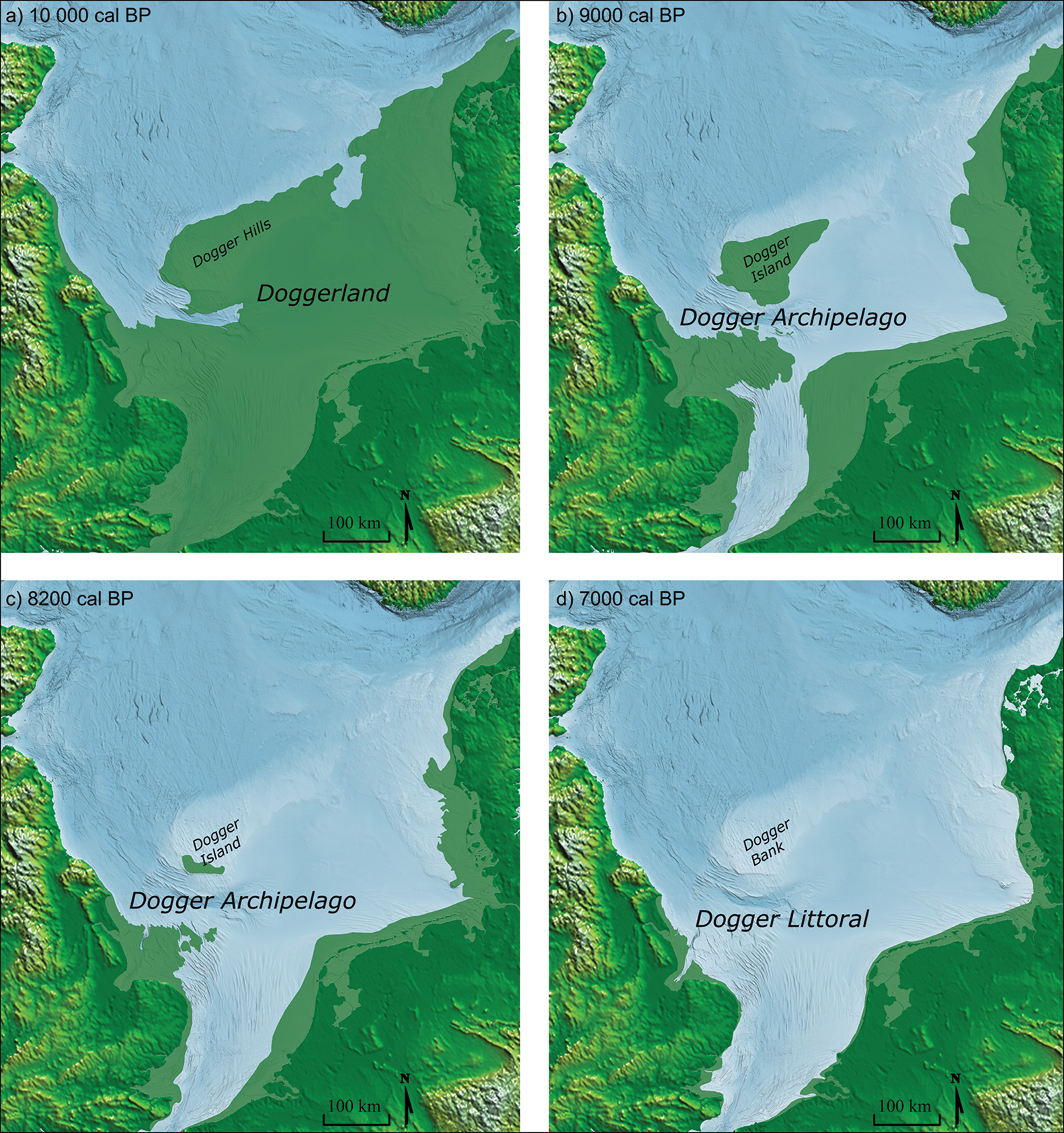
Figure 2. North Sea coastline reconstructions for: a) Doggerland c. 10 000 cal BP; b) Dogger Archipelago c. 9000 cal BP; c) Dogger Archipelago c. 8200 cal BP; d) Dogger Littoral c. 7000 cal BP (image by M. Muru).
Doggerland and the Dogger Hills
The extent of Doggerland during the Late Pleistocene is debated. Early Holocene Doggerland probably constituted a landscape stretching from Yorkshire to Denmark, with the Dogger Hills as a modest upland zone at its northernmost limit (Figure 2a). Subsequently, however, Doggerland became increasingly diminished and fragmented (Cohen et al. Reference Cohen, Westley, Erkens, Hijma, Weerts, Flemming, Harff, Moura, Burgess and Bailey2017: 162).
The Dogger Archipelago and Dogger Island
By 9000 cal BP, Doggerland would have been fragmented to the degree that it no longer resembled the continuous landscape often portrayed in the literature (e.g. Coles Reference Coles1998). The uplands, comprising the current Dogger Bank, would probably have been cut off (Figure 2b), forming Dogger Island, which survived for approximately another millennium. The rapidity of sea-level rise around this time (Hijma & Cohen Reference Hijma and Cohen2010) complicates assessment of the period for which this island remained an attractive ecological zone for exploitation or viable settlement. The broader landscape became increasingly fragmented by the formation of estuaries, inlets and islands (Gearey et al. Reference Gearey, Hopla, Boomer, Smith, Marshall, Fitch, Griffiths, Tappin, Williams, Hill, Boomer and Wilkinson2017: 49). From this period onwards—and certainly following the significant sea-level rise associated with the 8.2 ka event—it would be better to conceive of this landscape as the ‘Dogger Archipelago’.
The Dogger Littoral
Between 8400 and 8200 cal BP, the global average sea level rose (possibly in two phases) between 1 and 4m (Hijma & Cohen Reference Hijma and Cohen2019: 83). By the time the tsunami struck, c. 8150 BP, this higher sea level had probably reduced Dogger Island to a shallow sand bank (Hijma & Cohen Reference Hijma and Cohen2010, Reference Hijma and Cohen2019; Törnqvist & Hijma Reference Törnqvist and Hijma2012; Emery et al. Reference Emery, Hodgson, Barlow, Carrivick, Cotterill, Mellett and Booth2019). Reconstructing the contemporaneous shoreline using bathymetry and the relative sea-level curve (Shennan et al. Reference Shennan, Bradley and Edwards2018) indicates the possible survival of a small area (approximately 1000km2), comprising the highest terrain, south of the present-day sand bank (Figure 2c)—even assuming the maximal estimate of a 4m sea-level rise (Emery et al. Reference Emery, Hodgson, Barlow, Carrivick, Cotterill, Mellett and Booth2019). Many of the smaller islands to the south may also have remained above water (Peeters et al. Reference Peeters, Murphy and Flemming2009: 21; Garrow & Sturt Reference Garrow and Sturt2011: 63).
Around 7000 cal BP, approximately 1150 years after the tsunami, Dogger Island would presumably have disappeared and the number of islands reduced to a handful (Figure 2d). The shoreline of Denmark, north-west Germany, the Netherlands, Belgium and southern Britain continued to exist someway beyond their present extents, in some cases, such as the Wash in East Anglia, by several tens of kilometres. This is evidenced by the use of such coastal margins well into the Neolithic and later—as demonstrated by sites such as Seahenge off the UK coast and the many Late Mesolithic sites of the Danish nearshore. By this point, the remnants of Doggerland would cumulatively have still constituted a sizeable area: the ‘Dogger Littoral’ (Figure 2d).
Archaeological impact models
With a detailed understanding of the evolution of the landscape in place, competing models of the tsunami's impact may now be assessed. Two models relate to the impact on (extant) terrestrial landscapes (Waddington & Wicks Reference Waddington and Wicks2017; Blankholm Reference Blankholm2020), while two others concern the inundated southern North Sea landscape (Weninger et al. Reference Weninger2008; Hill et al. Reference Hill, Collins, Avdis, Kramer and Piggott2014, Reference Hill, Avdis, Mouradian, Collins and Piggott2017).
‘Doggerland’ wipeout scenario
To estimate the impact of Storegga, Weninger and colleagues (Reference Weninger2008) used dated sediments from Norway, Britain and Greenland that relate stratigraphically to tsunami deposits, along with bathymetric 3D modelling of the southern North Sea. They concluded that the Dogger Bank was probably submerged at the time of the tsunami, meaning that the wave may have reached the northern shores of present-day lowland Europe (Weninger et al. Reference Weninger2008). Without the barrier of the Dogger Bank, they argue that the Dogger Archipelago would have experienced devastating inundation with “a catastrophic impact on the contemporary coastal Mesolithic population” (Weninger et al. Reference Weninger2008: 17).
Dogger Bank survival scenario
An alternative scenario is advanced by Hill et al. (Reference Hill, Avdis, Mouradian, Collins and Piggott2017) using multiscale numeric modelling. Assuming the Dogger Bank to still have been both exposed and inhabited by Mesolithic communities, they initially proposed that the tsunami may have been catastrophic (Hill et al. Reference Hill, Collins, Avdis, Kramer and Piggott2014), but subsequently revised this position, concluding that a maximal inundation would have covered around 35 per cent of the exposed landmass—potentially similar to an extreme high tide (Hill et al. Reference Hill, Avdis, Mouradian, Collins and Piggott2017: 10). The authors, however, do not consider the presence of other landforms, and the pre-Storegga submergence of much of Dogger Island (Emery et al. Reference Emery, Hodgson, Barlow, Carrivick, Cotterill, Mellett and Booth2019) challenges this position.
Terrestrial models
The loss of coastline south of the isostatic readjustment margin since Storegga means that much currently extant landmass may be peripheral to the areas worst affected. Unlike sites along the Scottish and Norwegian coast, areas affected by the tsunami in the southern North Sea basin are likely to be under water, making it difficult to evaluate impact. Two recent studies, however, present archaeological evidence from north-east Britain and northern Norway for the terrestrial impact of the tsunami (Waddington & Wicks Reference Waddington and Wicks2017; Blankholm Reference Blankholm2020).
Waddington and Wicks (Reference Waddington and Wicks2017: fig. 4) observe a drop in the number of sites on the north-east coast of Britain prior to the tsunami, with a slow population rebound centred at c. 8000 cal BP. They argue that this is due to the tsunami scouring recently deposited archaeological remains from the Mesolithic coast. Although this interpretation is difficult to verify, subduction-based tsunami modelling suggests that scouring is strongest during the drawdown stage of a tsunami (Yeh & Li Reference Yeh, Li and Sekiguchi2008: 100), prior to the wave breaking on land. Furthermore, there appear to be no discernible changes in settlement type or artefacts associated with the time of the tsunami, although Waddington and Wicks (Reference Waddington and Wicks2017: 708) argue that this supports an externally induced drop in site density.
Typically, tsunami deposits rest unconformably on underlying strata, and are interpreted as being indicative of erosive events (Dawson Reference Dawson, Smith and Long1994: 88). It is, however, difficult to ascertain the severity of the erosion and whether it was sufficient to destroy or obscure centuries of human activity. Following the December 1992 tsunami that hit Flores in Indonesia, a conspicuous relationship between run-up height and erosion was observed. The maximal inundation distance for this tsunami, recorded along one river valley, was approximately 600m, but where run-up heights ranged between 1 and 4m along the northern coastal line of Flores, erosion was restricted to a narrow coastal strip (Shi & Smith Reference Shi and Smith2003: 191). Storegga run-up heights recorded from the UK mainland rarely exceed 4m, and would probably only surpass 5m in inlets and channels where wave energy could be focused (Smith et al. Reference Smith2004), especially south of the Forth estuary (Long Reference Long, Scourse, Chapman, Tappin and Wallis2018: 150).
While the Flores tsunami provides a rare example where it has been possible to investigate this relationship, it is not necessarily a suitable analogue. For Storegga, run-up heights—as inferred from field observations—are widely considered to represent minimal estimates (Dawson Reference Dawson, Smith and Long1994: 88), and may actually have been metres higher (Dawson Reference Dawson, Smith and Long1994; Smith et al. Reference Smith2004; Long Reference Long, Scourse, Chapman, Tappin and Wallis2018). Onshore tsunami geomorphology remains relatively poorly understood (although see Engel et al. Reference Engel, Pilarczyk, May, Brill and Garrett2020 for recent advancements), and wave run-up and backwash both have significant potential for surficial erosion (Sugawara et al. Reference Sugawara, Minoura, Imamura, Shiki, Tsuji, Yamazaki and Minoura2008). Ultimately, however, onshore wave velocities and, therefore, processes of sediment transportation and reworking will have been contingent upon local coastal topography (Dawson & Shi Reference Dawson and Shi2000; Gaffney et al. Reference Gaffney2020).
The Norwegian Varangerfjord study comprises a smaller area that is unrelated to the southern North Sea basin, and further removed from the location of the slides than north-east Britain. Varangerfjord is a relatively well protected, east-facing inlet with peninsulas to the west (Corner et al. Reference Corner, Yevzerov, Kolka and Møller1999: 147). Like Waddington and Wicks (Reference Waddington and Wicks2017), Blankholm (Reference Blankholm2020) notes a drop in the number of sites, but, in the absence of well-dated deposits, he is restricted to correlating this with elevation (26–28m asl), approximately 2–3m above the 8100 BP shoreline. Furthermore, there is no clear geological signature for the tsunami in this region, perhaps because it simply did not obtain a significant run-up height. Consequently, it is questionable to what extent the tsunami had an impact here (Blankholm Reference Blankholm2020).
Review of models
Neither the Varangerfjord nor UK models present compelling evidence of forced cultural change (cf. Torrence & Grattan Reference Torrence, Grattan, Torrence and Grattan2002) through conventional forms of material culture. Blankholm (Reference Blankholm2020) advocates caution, given the limited available data and potential for alternative ethnoarchaeological and taphonomic explanations for the site distribution patterns observed. Waddington and Wicks (Reference Waddington and Wicks2017), however, believe that the paucity of sites contemporaneous with—and centuries before—the tsunami results from the erosive force of its run-up.
The work of Weninger et al. (Reference Weninger2008) represents a tour de force of archaeological inference, exploring effects of a severe inundation in a way not previously attempted. Their assessment, however, does not consider the shoaling effect of the Dogger Bank. After reducing speed and increasing in amplification while passing over the shallow bank, wave energy would have dissipated upon re-entering deeper waters to the south. Furthermore, the rising waters were probably anything but “inexorable” (Weninger et al. Reference Weninger2008: 16). Tsunamis, like normal waves, break and then subside. Consequently, even following catastrophic inundation, the effect would not necessarily have led to permanent inundation. Although the tsunami may have devastated the remnant Dogger Island, it remains uncertain whether it was inhabited at this time. If it was, the hilly local topography may have offered some shelter from the impact.
Finally, although Hill et al.'s (Reference Hill, Collins, Avdis, Kramer and Piggott2014) model is not fully applicable—as Dogger Island was probably considerably smaller than posited when the tsunami struck (Figure 2c)—it nevertheless provides insight into how Storegga may have affected other low-lying landmasses. Even in a low-drag scenario with conservative wave-energy dissipation, it still predicts the survival of Dogger Island (Hill et al. Reference Hill, Avdis, Mouradian, Collins and Piggott2017)—without taking into account the effects of any extant vegetation cover (for a discussion of the arboreal effects on dissipation and inundation, see Cochard Reference Cochard2011).
Modern-day risk assessment models
Concerns about a future repeat of Storegga have prompted modern risk assessments (Chacón-Barrantes et al. Reference Chacón-Barrantes, Rangaswami and Mayerle2013). One model that focuses on the Dutch coast relocates the origin point of the tsunami to the entrance of the Norwegian trench, in order to provide a ‘maximal credible event’ scenario (Kulkarni et al. Reference Kulkarni, Zimmerman, Lanckriet, Breugem, Dorfmann and Zenz2017). Despite its fast rate of travel, the simulated tsunami failed to either breach or overtop the protective dune system on the Dutch foreshore, other than at its natural openings. Even here, waves failed to penetrate a second dune-line, and reached no more than 1km inland (Kulkarni et al. Reference Kulkarni, Zimmerman, Lanckriet, Breugem, Dorfmann and Zenz2017). The peak run-up was 7.5m, and the maximum inundation depth was 3.5m in a single, highly localised dune breach (Kulkarni et al. Reference Kulkarni, Zimmerman, Lanckriet, Breugem, Dorfmann and Zenz2017: 28). Furthermore, the run-up for the North Sea basin to the south of the Dogger Bank may have been less: the Storegga run-up heights (up to 5m) observed from south of the Forth estuary typically decrease in height farther south along the British coastline (Long Reference Long, Scourse, Chapman, Tappin and Wallis2018: fig. 6).
Variable run-up heights and sedimentation thicknesses attest to the importance of local topography in influencing the effects of coastal, wave-derived phenomena. Dawson et al. (Reference Dawson, Dawson, Bondevik, Costa, Hill and Stewart2020) attribute discrepancies between field observations and numerical simulations of run-up heights from the Shetland Islands to the influence of incising inlets and valleys. Even microtopography can have a significant influence. A winter storm surge in the southern North Sea in 2013, for example, produced run-up heights that varied by as much as 2m in the same locale at Holkham in Norfolk (Spencer et al. Reference Spencer, Brooks, Möller and Evans2014). This, combined with the highly localised inundation effects modelled by Kulkarni et al. (Reference Kulkarni, Zimmerman, Lanckriet, Breugem, Dorfmann and Zenz2017), and the caution that Mesolithic hunter-gatherers may have exercised to avoid danger zones (Leary Reference Leary2015: 80; Blankholm Reference Blankholm2020), suggests that we may need to revise our perception of Storegga as being universally devastating: a catastrophe for one group may be a ‘near miss’ for another.
New data from Doggerland
The influence of topography on the destructive potential of tsunamis has recently been affirmed by the recovery of the first evidence for the Storegga tsunami from the southern North Sea (Gaffney et al. Reference Gaffney2020). In 2018, a series of vibrocore samples were taken from palaeo-river channel systems associated with the Outer Dowsing Deep, as part of the ERC-funded project ‘Europe's Lost Frontiers’ (Gaffney et al. Reference Gaffney2020). Several cores contained ‘tsunami-like’ deposits, comprising clastic sediments, stones and broken shells, evincing a much higher level of turbation and accelerated deposition than the laminar sediments that bracket them. Multiproxy analyses of core ELF001A (Figure 3), including sedimentology, palaeomagnetic, isotopic, palaeobotany and sedaDNA techniques, confirm this assessment. Optically stimulated luminescence (OSL) dating sequences indicate that these deposits were broadly contemporaneous with Storegga (see Gaffney et al. Reference Gaffney2020; Figure S1 & Table S2).
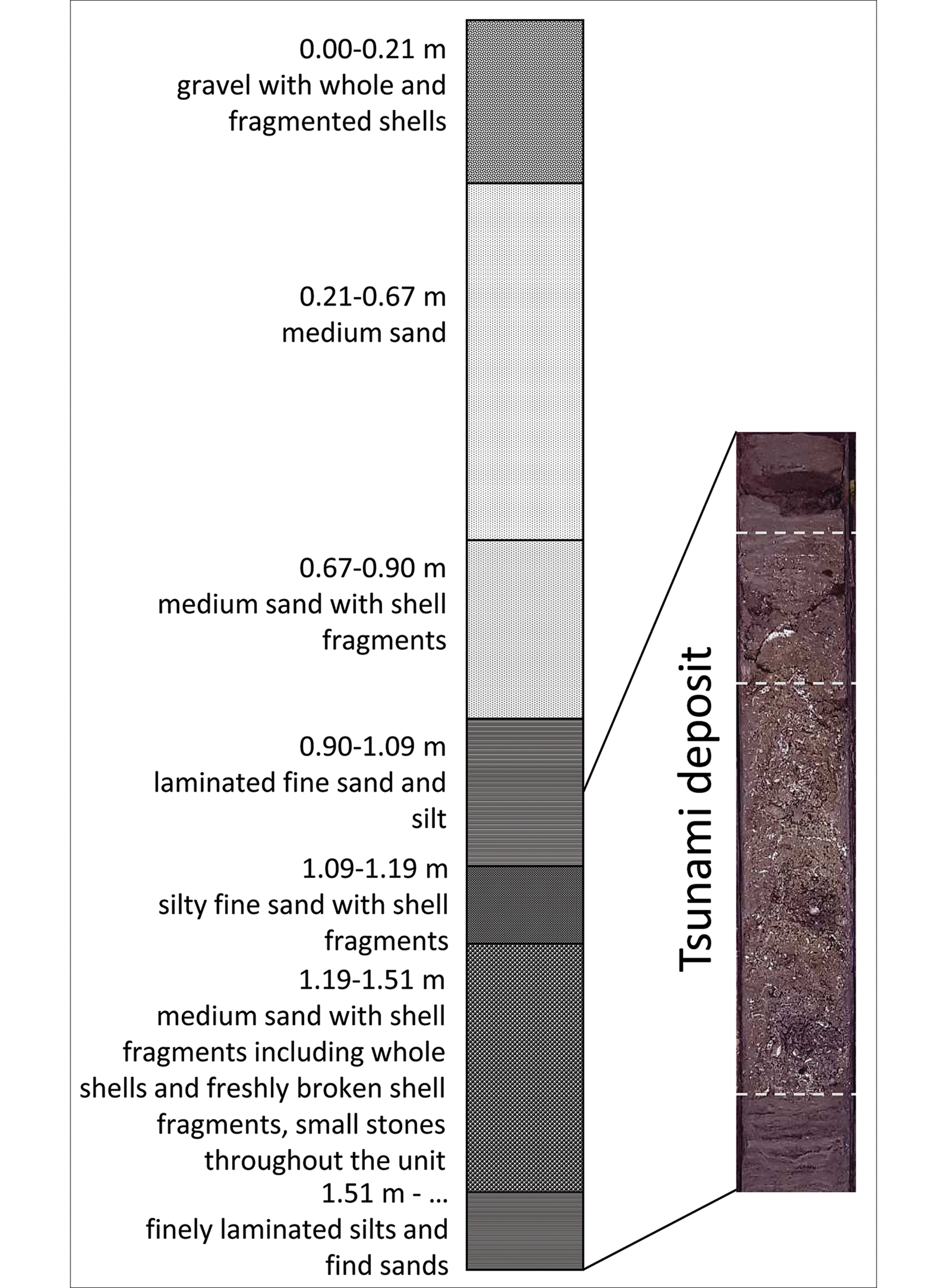
Figure 3. Stratigraphic units in core ELF001A (for full details, see Figure S1 & Table S2 in the online supplementary material; image by M. Muru & M. Bates).
The location of these deposits—42km inland from the present coastline (Figure 1b)—is striking, and their absence from other nearby cores reflects the importance of valleys and inlets in channelling wave-energy (Smith et al. Reference Smith2004; Gaffney et al. Reference Gaffney2020). Seismological mapping shows that, during the Early Holocene, the Dogger Bank (Cotterill et al. Reference Cotterill, James, Forsberg, Tjelta, Carter and Dove2017), along with other areas of Doggerland (Gaffney et al. Reference Gaffney, Thomson and Fitch2007), would have featured numerous fluvial-cut and glacial-infilled features that would have allowed for a highly variable inflow of water. The tsunami wave run-up in the area around core ELF001A can be estimated using bathymetry and the altitude of tsunami deposits within the core (Figures 3–4; Gaffney et al. Reference Gaffney2020). The remnant of Dogger Island may have acted as a physical barrier to the wave for areas to the south. The remaining submerged areas would have been particularly shallow at the time of the tsunami, and may have sheltered some areas to the south by causing the wave to prematurely shoal and dissipate, as seen in the Dogger Bank's influence on diurnal tides today (e.g. Pingree & Griffiths Reference Pingree and Griffiths1982). This shallow bank or low-lying island may, however, also have exacerbated the impact of the tsunami in other areas, focusing wave energy to the east and west of the bank, including the head of the Outer Dowsing Deep and the basin from which core ELF001A was retrieved (Figure 4).
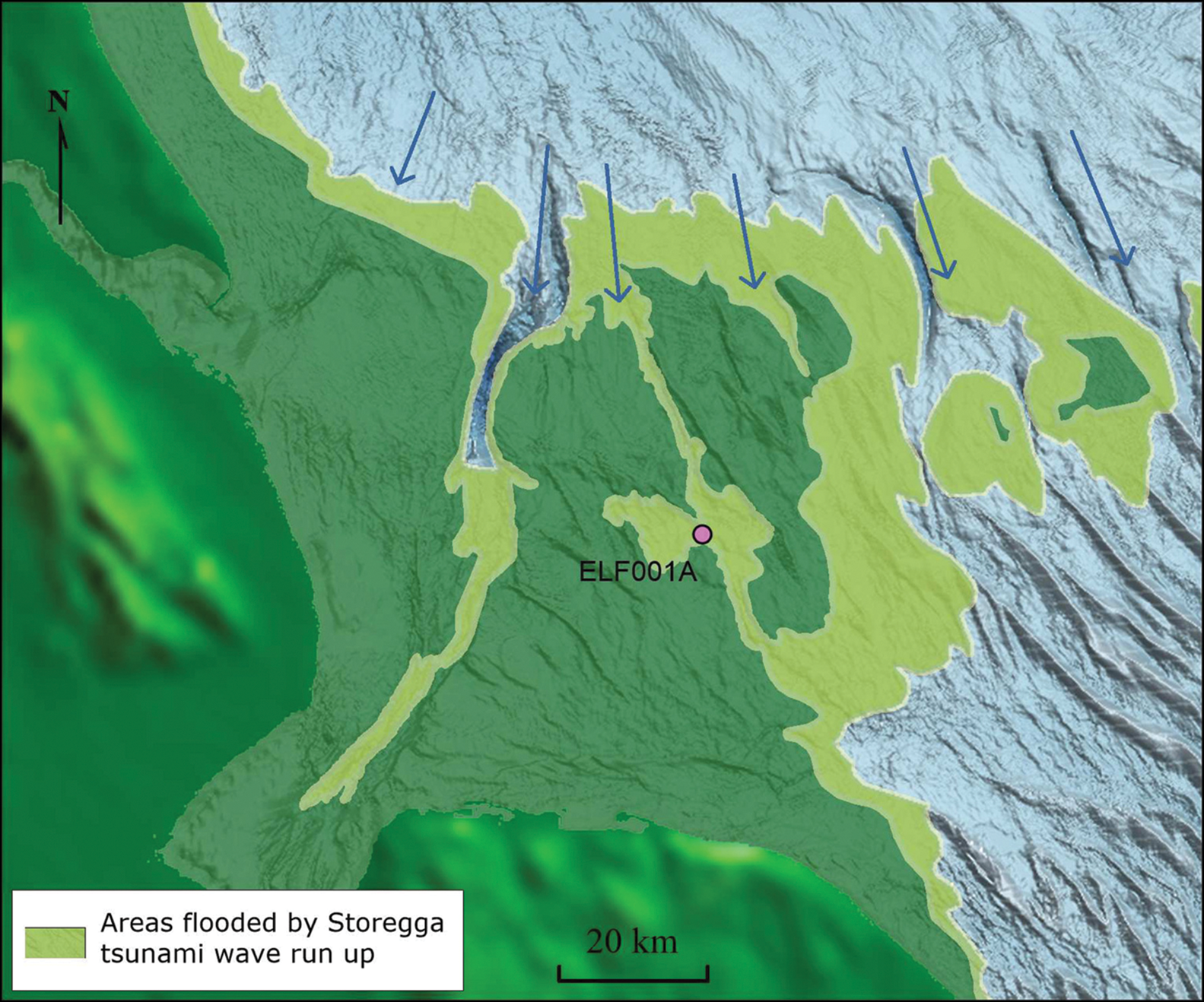
Figure 4. Model showing the Storegga tsunami and run-up around the western sector of the southern North Sea at 8150 cal BP (image by M. Muru).
Notably, sedaDNA evidence from the cores (Gaffney et al. Reference Gaffney2020) suggests a withdrawal of floodwaters and recovery of the land on the Dogger Littoral. Hence, the eventual inundation of the remaining parts of Doggerland resulted from the inexorable sea-level rise, rather than a lasting inundation from the Storegga tsunami.
Discussion
The Storegga tsunami was undoubtedly devastating for some regions. What was left of Dogger Island may have been particularly badly affected. The inlets and coastal valleys on the plains west of the Dogger Bank, however, may have been among the worst affected areas. In other locations, including areas protected by the Dogger Bank/Island, the impact may have been relatively limited. Nevertheless, the effects on any Mesolithic communities inhabiting the southern North Sea landscape remain, at present, difficult to gauge. As localised topographical variability is brought into focus, however, it seems increasingly untenable to maintain scenarios of wholesale catastrophic destruction or the definitive inundation of the last vestiges of Doggerland.
The prospect of the Dogger Littoral surviving the tsunami carries important implications for the prehistory of North-west Europe. The potential continuity of remnants of Doggerland into the Neolithic—as first proposed by Coles (Reference Coles, Coles, Coles and Jørgensen1999)—has been neglected due to the popularity of the notion of a catastrophic final inundation. It is typically assumed that ‘Doggerland’ had all but disappeared by the onset of the Neolithic (Cohen et al. Reference Cohen, Westley, Erkens, Hijma, Weerts, Flemming, Harff, Moura, Burgess and Bailey2017: 169). In the final stages of the European Mesolithic, hunter-gatherers formed a cultural, if not territorial, buffer between the incoming Linear Pottery Culture and the coasts. Increasingly, it seems that the spread of the Neolithic across North-west Europe was a rapid and stochastic affair (Rowley-Conwy Reference Rowley-Conwy2011), and the Dogger Littoral may have formed an exciting staging ground for whatever adaptations, innovations and social tensions comprised the final transition to farming (Garrow & Sturt Reference Garrow and Sturt2011).
Conclusion
The wealth of sedimentological evidence relating to the Storegga tsunami from around the northern North Sea basin makes the lack of archaeological evidence for the event even more curious. It seems reasonable to suggest that the Storegga tsunami must have been catastrophic to those caught within the run-in zone, and the event may have had significant knock-on effects for communities farther inland. Recent advances in palaeotopography, hydrological modelling and, now, the first evidence of the tsunami itself from the southern North Sea (Gaffney et al. Reference Gaffney2020) suggest that the impact of the tsunami would have been contingent upon regional variations in landscape and environment; this may begin to explain the puzzling absence of archaeological evidence.
Ultimately, the Storegga tsunami was neither universally catastrophic, nor was it a final flooding event for the Dogger Bank or the Dogger Littoral. The impact of the tsunami was highly contingent upon landscape dynamics, and the subsequent rise in sea level would have been temporary. Significant areas of the Dogger Littoral, if not also the Archipelago, may have survived well beyond the Storegga tsunami and into the Neolithic, a possibility that contributes to our understanding of the Mesolithic–Neolithic transition in North-west Europe.
Acknowledgements
We thank both Stein Bondevik and Marc Hijma for kindly sharing their work. We acknowledge PGS (https://www.pgs.com/) for provision of data used in this paper, under licence CA-BRAD-001-2017.
Funding statement
The study was supported by European Research Council funding through the European Union's Horizon 2020 research and innovation programme (project 670518 LOST FRONTIERS, https://erc.europa.eu/ https://lostfrontiers.teamapp.com/) and the Estonian Research Council grant (https://www.etag.ee; project PUTJD829).
Supplementary material
To view supplementary material for this article, please visit https://doi.org/10.15184/aqy.2020.49