Introduction
Margarosanite, ideally PbCa2Si3O9, was first described and named by Ford and Bradley (Reference Ford and Bradley1916), from the Parker Shaft in the Franklin Zn deposit, New Jersey, USA. Although it is an extremely rare mineral, Flink (Reference Flink1917) shortly thereafter reported its second occurrence, from the Långban Mn–Fe deposit, Värmland County, Sweden. Margarosanite was later also found at the nearby Harstigen and Jakobsberg mines. Anthropogenic margarosanite occurs on corroded lead bullets (Mera et al., Reference Mera, Rubio, Pérez, Galván and Germanier2015). Further studies on Franklin type specimens clarified the crystallography (Armstrong, Reference Armstrong1963) and the crystal structure of the mineral (Freed and Peacor, Reference Freed and Peacor1969).
Walstromite, BaCa2Si3O9, was first described by Alfors et al. (Reference Alfors, Stinson, Matthews and Pabst1965) from the Big Creek–Rush Creek area, Fresno County, California, USA. It was later reported from several other deposits in Western North America (Dunning et al., Reference Dunning, Walstrom and Lechner2018) and also from pyrometamorphic rocks (paralavas) of the Hatrurim Formation, Israel (Krzątała et al., Reference Krzątała, Krüger, Galuskina, Vapnik and Galuskin2020). Dent Glasser and Glasser (Reference Dent Glasser and Glasser1968) determined the crystal structure of synthetic walstromite, confirmed subsequently by Barkley et al. (Reference Barkley, Downs and Yang2011) using material from the type locality.
The isostructurality of margarosanite and walstromite was first postulated by Glasser and Glasser (Reference Glasser and Glasser1964) and subsequently confirmed by several authors (Dent Glasser and Glasser, Reference Dent Glasser and Glasser1968; Freed and Peacor, Reference Freed and Peacor1969; Callegari and Boiocchi, Reference Callegari and Boiocchi2016; Krzątała et al., Reference Krzątała, Krüger, Galuskina, Vapnik and Galuskin2020).
The Ca equivalent, CaCa2Si3O9, has been synthesised under high pressure and characterised (Trojer, Reference Trojer1969). The presence of Ca-silicate phases with margarosanite–walstromite structure type as inclusions in diamonds (Joswig et al., Reference Joswig, Stachel, Harris, Baur and Brey1999; Stachel et al., Reference Stachel, Harris, Brey and Joswig2000; Nasdala et al., Reference Nasdala, Brenker, Glinnemann, Hofmeister, Gasparik, Harris, Stachel and Reese2003; Brenker et al., Reference Brenker, Vincze, Vekemans, Nasdala, Stachel, Vollmer, Kersten, Somogyi, Adams, Joswig and Harris2005; Woodland et al., Reference Woodland, Girnis, Bulatov, Brey and Höfer2020) indicate a potentially Ca-rich lithology in the Earth's deep mantle. The isostructural, high-pressure form of CaCa2Si3O9, breyite (Brenker et al., Reference Brenker, Nestola, Brenker, Peruzzo and Harris2021), was discovered as inclusions in a super-deep diamond from the São Luiz placer deposits, Juína, Mato Grosso, Brazil.
The definition of the margarosanite group, presently including the three isostructural members margarosanite, walstromite and breyite, was approved by the Commission on New Minerals, Nomenclature and Classification (CNMNC) of the International Mineralogical Association (IMA) in June 2020 (Memorandum 96–SM20, Miyawaki et al., Reference Miyawaki, Hatert, Pasero and Mills2020). The proposal to the IMA and the present contribution were instigated by a find of intermediate mineral compositions in the walstromite–margarosanite series, in samples from the Jakobsberg deposit.
Description of walstromite-bearing skarn from the Jakobsberg deposit
The ores of the Jakobsberg deposit (59.83°N, 14.11°E), 1 km southeast of Nordmark, Filipstad, Värmland, Sweden, form separate hausmannite and magnetite–hematite bodies along with characteristic commonly banded skarn masses (Magnusson, Reference Magnusson1929). Modest amounts of Mn were mined from here 1864–1918, amounting to an equivalent of ~ 200 tons of extracted metal (Tegengren, Reference Tegengren1924). It is particularly known for exotic mineral assemblages of Pb silicates (Dunn et al., Reference Dunn, Peacor, Valley and Randall1985; Charalampides and Lindqvist, Reference Charalampides and Lindqvist1988; Holtstam and Langhof, Reference Holtstam and Langhof1994) and ferrites (Holtstam, Reference Holtstam1994; Holtstam et al., Reference Holtstam, Norrestam and Sjödin1995). Jakobsberg belongs to the so-called Långban-type localities (Moore, Reference Moore1970), carbonate-hosted Fe–Mn–(Ba–Pb–As–Sb) deposits in the Palaeoproterozoic Bergslagen ore region. They occur within supracrustal rock sequences dominated by ~1.9 Ga felsic metavolcanics that altogether have been regionally metamorphosed under mid amphibolite-facies conditions (500–650°C and 200–400 MPa) and to some extent also affected by Svecokarelian intrusive events in the region (Björck, Reference Björck1986). From a genetic point of view, the Långban-type ore deposits represent metamorphic analogues of shallow-marine, exhalative Mn–Fe deposits (Boström et al., Reference Boström, Rydell and Joensuu1979; Holtstam and Mansfeld, Reference Holtstam and Mansfeld2001).
The sample investigated (GEO-NRM catalogue #19940358) is a medium-grained silicate skarn, with subordinate baryte and native copper present. Carbonates and oxides are absent. The rock is exceedingly heterogeneous in terms of mineral composition, and irregularly banded, as clearly seen under short-wave ultraviolet light. Non-fluorescent parts mainly consist of ganomalite, phlogopite, vesuvianite and andradite. Bright orange-yellow fluorescent wollastonite crystals up to 5 mm occurs with mainly phlogopite and garnet. Minor amounts of ganomalite (low in MnO, ~1 wt.%, thus forming a near-midpoint solid solution with wayneburnhamite, Pb9Ca6(Si2O7)3(SiO4)3; Kampf et al., Reference Kampf, Housley and Rossman2016) are also found here.
A smaller portion of the rock (as 5–15 mm wide bands) is dominated by bluish-white (under ultraviolet) celsian, blue walstromite (i.e. the walstromite–margarosanite solid solution described here), phlogopite and Al-rich andradite. Walstromite forms subhedral, often lamellar crystals up to ca. 0.5 mm in longest dimension (Fig. 1), occasionally united in multigranular aggregates, sometimes showing a skeletal to poikiloblastic appearance, with inclusions of celsian. From the textural observations we conclude that phlogopite and celsian are the most primary constituents of the assemblage along with partially resorbed accessory andradite. Fluid-mediated remobilisation of Pb obtained from ganomalite or some other precursor Pb mineral might have led to formation of walstromite–margarosanite. Vesuvianite, Mn-rich diopside and sparse grossular also belong to this association. Nasonite occurs as grains up to 0.3 mm across, usually in direct contact with walstromite. On rims and along cracks and cleavage traces of walstromite, margarosanite with near-end member composition occurs as over- and intergrowths (Fig. 2), interpreted as a late pseudomorphic replacement. As can be seen in back-scatter electron (BSE) images with enhanced contrast, walstromite is sometimes heterogeneous, with a sector-like zonation linked to variations of Ba and Pb contents.

Fig. 1. SEM-BSE image of the mineral assemblage in sample GEO-NRM #19940358, with walstromite–margarosanite (Wal), celsian (Cls), phlogopite (Phl), andradite (Adr) and nasonite (Nas).

Fig. 2. SEM-BSE image, detail of Fig 1. Arrow points to a phase with margarosanite end-member composition (GEO-NRM catalogue #19940358).
Experimental
Single-crystal X-ray diffraction data were collected with a Rigaku Oxford Diffraction XtaLAB Synergy diffractometer equipped with a PhotonJet (Mo) X-ray Source operating at 50 kV and 1 mA, and a Hybrid Pixel Array detector at 62 mm from the sample position. A large number of images (27,030) were taken at 0.2° rotation of ω-rotations at 0.36 s per exposure in shutterless mode in order to cover a full sphere of the reciprocal space and to get accurate intensity data. The crystal structure was refined using SHELX-2018 (Sheldrick, Reference Sheldrick2015), starting from the atomic coordinates of Freed and Peacor (Reference Freed and Peacor1969). Neutral scattering curves were employed and anisotropic displacement parameters were refined. Crystal and refinement data are given in Table 1. Atomic coordinates, equivalent displacement parameters, and site occupancies are reported in Table 2, whereas selected bond distances and angles obtained are found in Table 3. The crystallographic information file has been deposited with the Principal Editor of Mineralogical Magazine and is available as Supplementary material (see below).
Table 1. Data and experimental details for single-crystal X-ray diffraction study of Pb-rich walstromite from Jakobsberg.
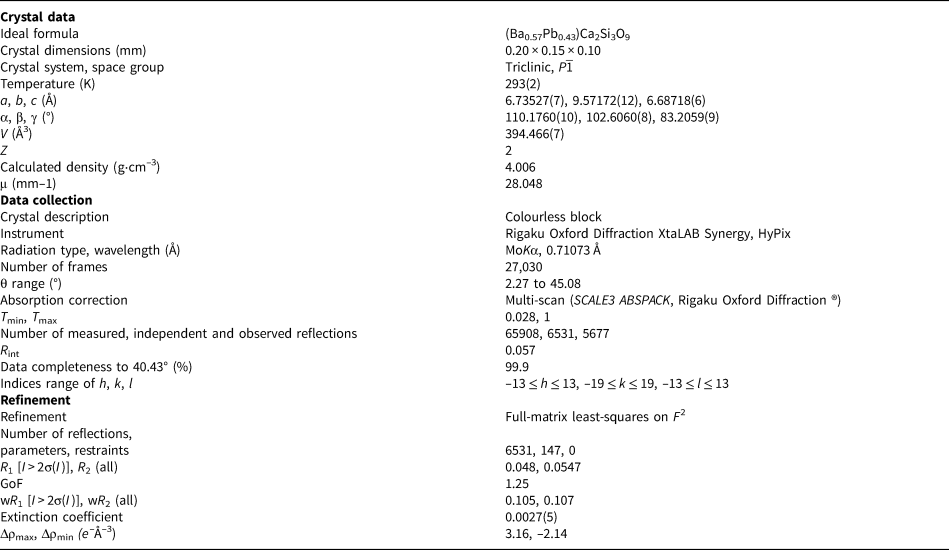
Table 2. Fractional atomic coordinates, thermal parameters and occupancies.

Occupancies: Ca3a = 0.570(4); Ca3b = 0.430(4)
Table 3. Selected interatomic distances (Å), angles (°), tetrahedral angle variances (TAV, °2) and quadratic elongations (TQE) according to Robinson et al. (Reference Robinson, Gibbs and Ribbe1971) for walstromite–margarosanite.

Chemical data were obtained through an FEI Quanta 650 field-emission scanning electron microscope (SEM) fitted with an 80 mm2 X-MaxN Oxford Instruments energy-dispersive spectroscopy (EDS) detector (accelerating voltage 20 kV, beam size 1 μm and working distance 10 mm). Beam current was calibrated on Co metal, with the instrument calibrated against reference materials for each element (quartz for Si; wollastonite for Ca, BaF2 for Ba, PbTe for Pb and pure metal for Mn). Chemical data are available in supplementary material, Table S1.
Micro-Raman measurements were performed using a Horiba (Jobin Yvon) LabRam HR Evolution. Polished sample surfaces were excited with an air-cooled frequency doubled 532 nm Nd-YAG laser utilising an Olympus 100× objective (NA = 0.9). Spectra were generated in the range of 1800 to 200 cm–1 utilising a 600 grooves/cm grating. The spectral resolution was in the order of 1 cm–1 and the lateral resolution was ~1 μm. The wavenumber calibration was done using the 520.7 cm–1 Raman band on a polished Si wafer with a wavenumber accuracy usually better than 0.5 cm–1. The spectra shown (Fig. 3) were collected through two acquisition cycles with single counting times of 45 s in a close to back-scattered geometry, on regions previously analysed during SEM studies.

Fig. 3. Raman spectra obtained with a 532-nm laser. Spectra are displaced 4000–6000 units on the Y axis for clarity.
Mineral chemistry
The sample from the Jakobsberg deposit displays variable Ba–Pb compositions in the range from ca. 50 mol.% margarosanite–50% mol.% walstromite to ca. 30 mol.% margarosanite–70 mol.% walstromite (Fig. 4). The Mn content is low and constant, 0.01–0.02 apfu. No other elements were detected.

Fig. 4. Chemical variation Ba vs. Pb in walstromite–margarosanite based on EDS analyses. The linear regression line (R 2 = 0.99) verifies the binary solid solution.
Electron probe microanalysis data in the literature show only minor compositional variations for these minerals. Margarosanite from Franklin includes 0.8 wt.% ZnO and 0.6 wt.% MnO (Dunn, Reference Dunn1985), whereas Långban samples could have up to 2.8 wt.% MnO and 0.2 wt.% BaO (Charalampides and Lindqvist, Reference Charalampides and Lindqvist1988). Walstromite from Fresno, California shows up to 0.4 wt.% SrO, 0.5% wt.% MnO (RRUFF no. R070634.2, Lafuente et al., Reference Lafuente, Downs, Yang, Stone, Armbruster and Danisi2015) and 0.3 wt.% Al2O3 (Basciano, Reference Basciano1999), whereas walstromite from Hatrurim contains up to 0.4 wt.% TiO2 and 0.7 wt.% SrO (Krzątała et al., Reference Krzątała, Krüger, Galuskina, Vapnik and Galuskin2020). Furthermore, there is no evidence for substitution between Ca2+ and the two larger cations Ba2+ and Pb2+ in walstromite or margarosanite, respectively. For breyite, no foreign cations are reported (Brenker et al., Reference Brenker, Nestola, Brenker, Peruzzo and Harris2021). Intermediate compositions between margarosanite and walstromite, indicating an extensive solid-solution series, have never been shown to exist up to now.
Raman spectra
The Raman spectrum of Pb-rich walstromite is affected by both a high fluorescence background (in particular above 800 cm–1) and broadening of peaks (Fig. 3). As expected, there is significant resemblance between the spectra of walstromite and of pure margarosanite (obtained from GEO-NRM #g38818, a Jakobsberg sample). The considerable broadening of the walstromite peaks can be explained by positional disorder induced by randomly distributed Pb/Ba cations; see also Alia et al. (Reference Alia, Edwards, Lopez-Andres, Gonzalez-Martin, Garcia-Navarro and Mansour2000).
The prominent Raman band at 654.5 cm–1 obtained for end-member margarosanite is related to Si–O–Si symmetric stretching vibrations in the 3-membered silicate rings, analogous to, e.g. the main band at ~580 cm–1 of benitoite and isostructural minerals (Takahashi et al., Reference Takahashi, Iwasaki, Masai and Fujiwara2008) and the corresponding band at 666 nm in roeblingite (Långban specimen, RRUFF ID: R140328, Lafuente et al., Reference Lafuente, Downs, Yang, Stone, Armbruster and Danisi2015). This particular band is shifted to 650–648 cm–1, and the peak position is sensitive to composition of the analysed spot (shifted to lower frequency with higher BaO contents). The bands at ca. 980–960 are possibly related to Si–O stretching vibrations. Distinct bands at ca. 576 and 493 cm–1 are common for all the spectra and due to bending vibrations in the silicate rings (Chukanov and Chervonnyi, Reference Chukanov and Chervonnyi2016). The intense 1010 cm–1 band of pure margarosanite has no defined counterpart in walstromite. End-member walstromite has a distinct band at 988 cm–1 (measured with a 633-nm laser; Gaft et al., Reference Gaft, Yeates and Nagli2013), whereas the present analyses of the Jakobsberg sample shows only a broad feature at ca. 975 cm–1.
Single-crystal structural study
The minerals of the margarosanite group are Ca-(Ba, Pb) cyclosilicates with three-membered [Si3O9]6– rings (3R) in layers with the apices of alternating rings pointing in opposite directions (Fig. 5a,b). The crystal structure can be described as a closest-packing of O atoms with compact layers parallel to (101), hosting Si and Ca (Ba, Pb) cations at interstitial sites. There are three unique Si sites in each ring, whereas Ca occupies two nonequivalent sites: Ca1 with 8-coordination to O; and Ca2 with 6-coordination. Ba2+ and Pb2+ reside in a unique 6+4 coordinated position (6+1 for Ca in breyite), within the 3R-layer in a peripheral position.
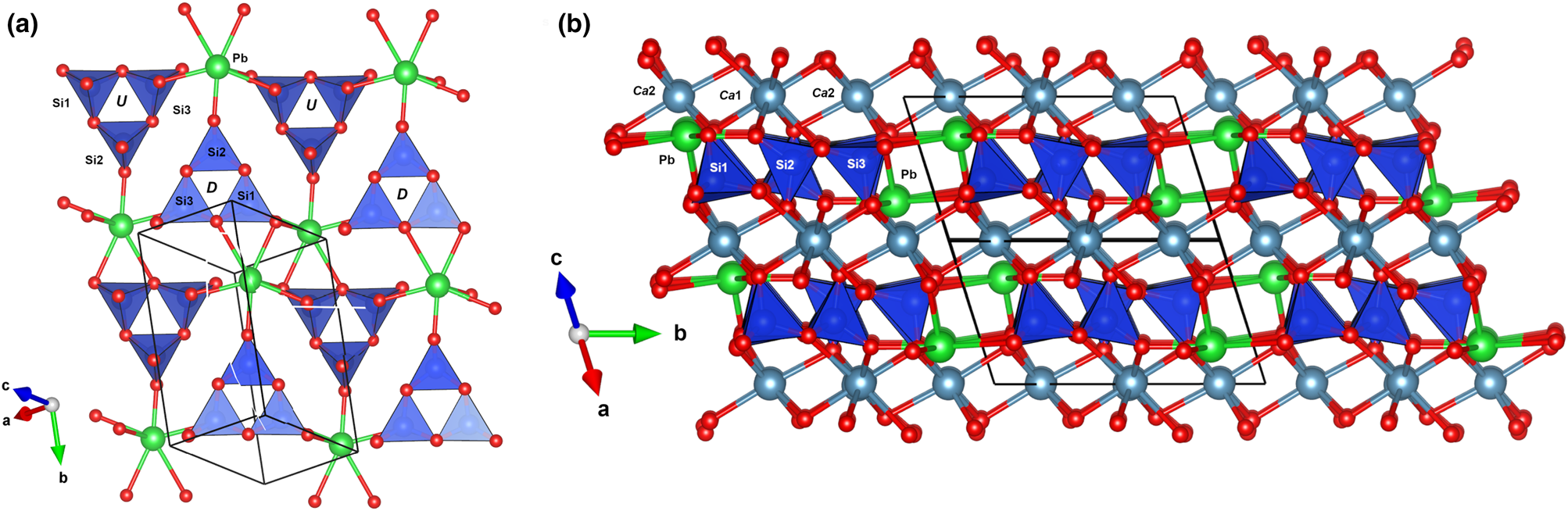
Fig. 5. The ‘layer’ of 3-membered rings of SiO4 tetrahedra in margarosanite (a) and the relative position of sites occupied by Ca (Ca1 and Ca2) and by Pb(Ba) (Ca3) (b). Figures obtained with Vesta 3.0 (Momma and Izumi, Reference Momma and Izumi2011).
Trojer (Reference Trojer1969) described the structure of ‘wollastonite-II’ as a high-pressure modification in a synthetic analogue. Later Joswig et al. (Reference Joswig, Paulus, Winkler and Milman2003) described ‘CaSiO3-walstromite’ and discussed the relationships with ‘wollastonite-II’. These authors reported that the axial Si3O9-ring could be isomorphously replaced by the same ring with the ‘inverse axiality’. A detailed study by Barkley et al. (Reference Barkley, Downs and Yang2011) showed that the topology is identical (also to walstromite) and that both structures are equivalent after an opportune transformation of coordinates using the matrix

The corresponding unit-cell parameters for wollastonite-II after the transformation are a = 6.695, b = 9.429, c = 6.666 Å, α = 83.671, β = 76.133 and γ = 67.639°. This CaSiO3 phase was subsequently thus named breyite (Brenker et al., Reference Brenker, Nestola, Brenker, Peruzzo and Harris2021), also corresponding to the ‘walstromite-structured CaSiO3’ (e.g. Joswig et al., Reference Joswig, Stachel, Harris, Baur and Brey1999; Brenker et al., Reference Brenker, Vollmer, Vincze, Vekemans, Szymanski, Janssens, Szaloki, Nasdala, Joswig and Kaminsky2007) or ‘CaSiO3-walstromite’ (Barkley et al., Reference Barkley, Downs and Yang2011; Anzolini et al., Reference Anzolini, Angel, Merlini, Derzsi, Tokár, Milani, Krebs, Brenker, Nestola and Harris2016).
The unit-cell parameters of all the members of the group, including our new data, are given in Table 4. Whereas those are all isotypic, each mineral has unfortunately been described with a different crystallographic setting. The Niggli cell for margarosanite is: a = 6.696, b = 6.739, c = 9.529 Å, α = 83.04, β = 69.81, γ = 76.95° and V = 392.75 Å3.
The setting used by Freed and Peacor (Reference Freed and Peacor1969) can be obtained from the Niggli cell using the matrix

Table 4. Chemical formulae and crystallographic data for margarosanite-group members (natural samples). Triclinic symmetry, space group P 1 and Z = 2.

1Callegari et al. (Reference Callegari and Boiocchi2016); 2Barkley et al. (Reference Barkley, Downs and Yang2011); 3Brenker et al. (Reference Brenker, Nestola, Brenker, Peruzzo and Harris2021); 4this work
The setting used for walstromite by Dent Glasser and Glasser (Reference Dent Glasser and Glasser1968) can be obtained from the Niggli cell using the matrix

The setting used for breyite by Brenker et al. (Reference Brenker, Nestola, Brenker, Peruzzo and Harris2021) can be obtained from the Niggli cell using the matrix

The 3R-topology of the rings of SiO4 tetrahedra is almost unique among minerals. Three-membered rings occur in ‘benitoite-group’ minerals (benitoite, bazirite and pabstite; informally grouped by Hawthorne, Reference Hawthorne1987) as well as in wadeite, calciocatapleiite and catapleiite. However, only in the rare complex Pb oxysalt roeblingite, Pb2Ca6(SO4)2(OH)2(H2O)4[Mn(Si3O9)2], do the SiO4 tetrahedra have exactly the same configuration as in margarosanite (Moore and Shen, Reference Moore and Shen1984). In margarosanite and roeblingite the tetrahedra in the 3R units point all up or all down (u3 or d3, following the Hawthorne et al., Reference Hawthorne, Uvarova and Sokolova2019 coding for nets) alternating in the same plane containing the 3R-units, whereas in the remaining above mentioned 3R-unit silicates the tetrahedra point neither up nor down (o3).
The crystal-structure analysis of the Jakobsberg specimen with composition ca. (Ba0.57Pb0.43)Ca2Si3O9 confirmed the structure type. In addition, it can be noted that the Ca3 (Ba, Pb) site is split into two positions separated by 0.392(2) Å, with the position occupied by Ba found slightly more peripheral to the 3R-layers (Fig. 6). Moore et al. (Reference Moore, Davis, Van Derveer and Gupta1993) compared the crystal structures of end-member margarosanite and walstromite and found a difference of 0.43 Å, ascribed to the stereochemically active 6s 2 lone electron pair of the Pb2+ ion. An analogous splitting of ~0.5 Å was found in the structure of hyalotekite (Ba,Pb,K)4(Ca,Y)2(B,Be)2(Si,B)2Si8O28F, Christy et al., Reference Christy, Grew, Mayo, Yates and Belakovskiy1998), a mineral described for the first time at Långban (Nordenskiöld, Reference Nordenskiöld1877).

Fig. 6. The Ca3 coordination polyhedron with shifted Pb atoms at the Ca3b site due to the lone pair effect of Pb2+. Figures obtained with Vesta 3.0 (Momma and Izumi, Reference Momma and Izumi2011).
Nomenclature
Minerals of the margarosanite group have the general formula AB 2Si3O9, where A (Ca3 site) = Ca, Ba and Pb and B (Ca1 and Ca2 sites) = Ca. Root names therefore depend on the composition at A. The presence of non-dominant amounts of cations at the Ca3 site are described with a modifier (Pb-, Ba- or Ca-rich).
Margarosanite clearly has priority in terms of historical precedence, but the structure type has become more familiar under a different name (walstromite). However, neither the ‘margarosanite group’ nor the ‘walstromite group’ seem to have been used as a term used in the past (except for the unapproved use by Krzątała et al., Reference Krzątała, Krüger, Galuskina, Vapnik and Galuskin2020). According to Mills et al. (Reference Mills, Hatert, Nickel and Ferraris2009), a group name can be selected contrary to the precedence rule when the name of this group is very firmly established in the literature. In this case, we found insufficient arguments to apply this rule of exception.
Further members of the group are possible in Nature. Substitution of Sr for Ca is known in synthetic compounds, such as Ca0.43Sr0.57[SiO3] (Dörsam et al. (Reference Dörsam, Liebscher, Wunder, Franz and Gottschalk2009) and Sr[SiO3] (Machida et al., Reference Machida, Adachi, Shiokawa, Shimada, Koizumi, Suito and Onodera1982), leading to the possible CaCa2Si3O9–SrSr2Si3O9 solid solution suggested by Barkley et al. (Reference Barkley, Downs and Yang2011). A Ba–Sr member seems possible, from the existence of isostructural synthetic BaSr2Ge3O9 (Bräuchle et al., Reference Bräuchle, Hejny and Huppertz2016). Phosphate structural analogues, e.g. KNa2P3O9 (Tordjman et al., Reference Tordjman, Durif and Cavero-Ghersi1974) are known as synthetic compounds. If they are discovered in geological environments, a mineral supergroup of the general formula AB 2T 3O9 should be defined. Interestingly, a high-pressure polymorph of Fe-rich dolomite, ‘dolomite-IV’, has a topologically similar crystal structure (with tetrahedrally coordinated C atoms; Merlini et al., Reference Merlini, Cerantola, Gatta, Gemmi, Hanfland, Kupenko, Lotti, Müller and Zhang2017).
Comment on Ba–Pb isomorphism
Ba-for-Pb replacement is very rare among silicate minerals, found only in the hyalotekite group (Hawthorne et al., Reference Hawthorne, Sokolova, Agakhanov, Pautov, Karpenko and Grew2018). Members of the feldspar group could potentially host large amounts of Pb, such as in the Pb-rich orthoclase from Broken Hill, NSW, Australia (e.g. Plimer, Reference Plimer1976). Celsian in the present sample has no Pb (at the EDS detection limit, ≥ 0.2 wt.% PbO). Celsian from Jakobsberg is shown to be dominantly Al–Si ordered, has the monoclinic I2/c structure (Griffin and Ribbe, Reference Griffen and Ribbe1976), and is essentially isostructural with ordered synthetic PbAl2Si2O8 (Benna et al., Reference Benna, Tribaudino and Bruno1996). There is no obvious reason why intermediate (Pb,Ba)Al2Si2O8 compositions should not exist in Nature, in the same fashion as for margarosanite–walstromite, provided that the appropriate bulk composition can be achieved. The most Ba–Pb-rich feldspar known is a hyalophane from the Långban deposit (Christy and Gatedal, Reference Christy and Gatedal2005). The effect of the Pb2+ lone pair in feldspar is displacive for the Pb atoms, and it changes the anion coordination from nominally 7-fold to an irregular 6-fold configuration (Benna et al., Reference Benna, Tribaudino and Bruno1996). Such phenomena could have an effect on the solid solubility, but are largely still unexplored. Margarosanite is stable to ~800°C at ambient pressure (Jak et al., Reference Jak, Hayes and Liu1998), whereas walstromite has an extended stability to at least 1200°C (Shukla et al., Reference Shukla, Jung, Decterov and Pelton2018). No data are available for intermediate compositions of the series. If a solvus exists in it, the corresponding immiscibility gap must lie below the maximum temperature of regional metamorphism, not greatly exceeding 650°C in the area.
Supplementary material
To view supplementary material for this article, please visit https://doi.org/10.1180/mgm.2021.15
Acknowledgements
Lennart Öhman (†) and Andreas Borsos called our attention to the blue-fluorescent mineral from Jakobsberg. F.C. acknowledges financial support by the grant “Ricerca Locale 2014”, Università di Milano, and by the grant from the Italian Ministry of Education (MIUR) through the project “Dipartimenti di Eccellenza 2018–2022”. Comments from two anonymous journal reviewers helped to improve the text.