INTRODUCTION
The NEIF Radiocarbon Laboratory (NRCL) at the Scottish Universities Environmental Research Centre focuses on exploring applications of radiocarbon within a broad definition of Earth and environmental science. The laboratory has been in existence since 1971 and hence builds upon a long experience in these applications. Since 2019 the laboratory has formed part of the National Environmental Isotope Facility (NEIF). This provides UK researchers with access to “an integrated platform of state-of-the-art isotope and organic geochemistry analytical capabilities and specialisms” (https://www.isotopesuk.org/). Researchers eligible for UK Research and Innovation funding can apply for measurements free-at-point-of-delivery from NRCL. Along with these activities, the laboratory performs collaborative research with a wide range of international groups, together with in-house innovation and development in the field of radiocarbon science.
The NRCL has capabilities for the analysis of a wide range of sample matrices (Table 1). In practice this covers studies for which radiocarbon is used to provide chronological information (e.g., palaeoenvironment, palaeoclimate, and palaeoceanography), and research in which radiocarbon is a valuable tracer of stocks, fluxes, residence times and turnover (e.g., cycling of carbon in systems such as soils, sediments, or freshwaters).
Table 1 Sample matrices upon which radiocarbon measurements are made at the NRCL.

Along with radiocarbon measurement of samples, the NRCL also engages in the design and build of novel equipment for field-collection of samples for radiocarbon analysis, laboratory experiments for radiocarbon sample collection (e.g., soil incubations), and the improvement or development of techniques, methods, and approaches for sample processing. Present throughput is ca. 2000 14C measurements per year by accelerator mass spectrometry (AMS), via the preparation of graphite from sample CO2. The typical size range of graphite prepared by the laboratory ranges down to c.100 µg carbon, with ca. 15–20% of samples in the size range 100–500 µg carbon, and the remainder >500 µg carbon.
Sample Pretreatment Methods and Generation of CO2 from Pretreated Samples
Below we describe procedures and methods used in the NRCL for processing of samples submitted to the laboratory. This covers both pretreatment and the subsequent generation and purification of CO2 from samples for stable carbon isotope measurement and graphitisation prior to 14C measurement. This discussion is ordered by the general categories listed in Table 1 for convenience.
All borosilicate glassware and metal tools used in chemical or physical pretreatment of samples are first precleaned by mechanical cleaning and washing in carbon-free detergent (Decon 90, Decon Laboratories Ltd, UK). This is followed by further mechanical cleaning, washing, and rinsing in MilliQTM water, and finally combustion in a muffle furnace at 450ºC for a minimum of two hours. All filter papers used in sample handling are either glass fiber or quartz fiber and are precleaned by combustion at 450ºC for two hours before use. All quartz glassware and chemicals (e.g., Ag, Cu, Zn, Fe) used in the preparation of sample CO2 and graphitisation are precleaned by combustion in a muffle furnace at 900ºC for two hours. All water used in sample preparation is ultrapure MilliQ™ deionized water. All chemicals used for wet chemistry during sample preparation are a minimum of “Analytical grade.”
Samples are usually processed in batches of ca. 10 individual samples, accompanied by at least one “process standard” (see Table 2). This is a known-age material that is matrix-matched to the chemical composition of the samples as far as practical. The choice of appropriate process standard(s) that are treated alongside the samples also considers the estimated 14C/12C isotopic composition of the sample materials, to provide the best possible quality assurance information that is tailored to the project in question. Further detail on quality assurance processes is provided below.
Table 2 Current materials used as process standards by NRCL.
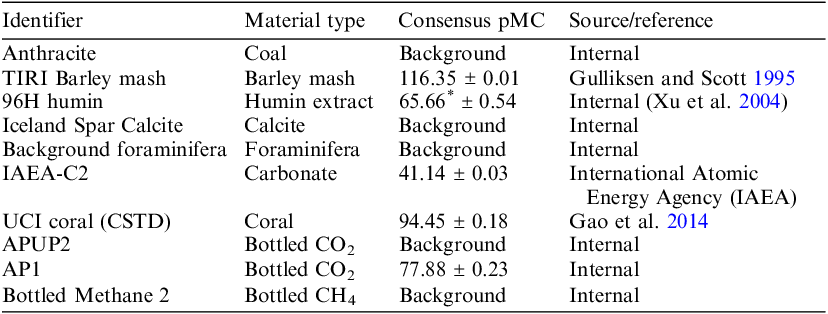
* Continual analysis of internal measurements has resulted in adjustment of this value to 65.63 pMC.
Organic Materials
Organic materials such as plant remains or charcoal (including individual seeds, nutshell, leaves etc.) are usually pretreated by acid-base-acid (ABA) methods. For the majority of samples this involves digestion in 1 M HCl (80ºC, 2 hours) to remove carbonates and some mobile contaminants (e.g., fulvic acids), after which the sample is washed free from mineral acid with MilliQTM water until neutral. This may be achieved by either filtration, or decanting, depending on the sample size and nature. For very small samples it may be necessary to remove the solution at each step of the ABA by pipetting. The sample is then digested in 0.5 M NaOH (80ºC, 2 hours) to remove alkali-soluble contaminants such as humic and other fulvic acids. The digestion is repeated until no further humic material is extracted (based on a fully clear solution), at which point the residue is rinsed free of alkali with MilliQTM water before a final digestion in 1 M HCl (80ºC, 1 hour). After this the sample is rinsed free of acid, dried and homogenized. For particularly delicate plant macrofossils a milder approach is adopted, where the first acid digestion is reduced to 30 minutes, and the alkali digestion is performed using 0.2 M NaOH for 20 minutes at 80ºC. After this the remainder of the treatment is identical to the standard ABA. Note that for some projects a preparatory step may be required prior to the ABA treatment commencing. This is considered on a project-specific and sample-specific basis. Examples include solvent washes to remove material such as waxes, oils, and other lipids that are regarded as contaminants relative to the target for measurement. For wood samples an ABA treatment is followed by a bleaching step with acidified sodium hypochlorite solution to isolate the wood carbohydrates (the dating target) from mobile organic fractions. Typically, this is performed at 70ºC and continues until isolation of carbohydrates is complete.
Other, more specialized, organic remains are pretreated by methods specific to the individual project. This may involve part, or all of the processes being performed at the submitter’s laboratory, for example in the case of pollen concentrates. In these instances, the NRCL provides a range of verified standard and blank materials (see Table 2) for processing in the submitter’s laboratory alongside the unknown samples, to monitor quality assurance through the entire process. This allows us to quantify the amount and 14C content of any carbon contaminants during any processes performed outside of the NRCL before sample submission for 14C measurement. It also allows a correction to be made to the measured sample 14C that accounts for the addition of exogenous carbon that is extra to that added during NRCL processing of samples.
After pretreatment, dried, solid organic remains are converted to CO2 via one of three methods. Primarily, we use the quartz tube combustion method as described in Vandeputte et al. (1996). Samples are placed in precleaned quartz tubes (QT) together with copper wires and silver foil. Smaller samples are inserted into the QT contained within quartz inserts. The tube is evacuated and sealed before combustion at 900ºC for 5 hours in a muffle furnace (CarboliteTM, UK). The second option is combustion in an Elemental Analyser (EA: Costech ECS4010, Italy). This is typically used for samples with a low carbon content, and/or a high content of compounds that would be problematic for quartz tube combustion. This approach is used to overcome issues such as sample sizes that would exceed the capacity of the quartz tubes, and risk breakage of the quartz tubes due to gas expansion during combustion. Pretreated samples are weighed into precleaned silver capsules and placed into an autosampler above the EA combustion furnace. Multiple combustions may be performed on a single sample within the combustion furnace in order to ensure that all sample CO2 has been released. The outlet of the EA is connected directly to a vacuum line for cryogenic purification and aliquoting. The third approach is by oxidation under high pressure pure oxygen in a combustion chamber (conventionally known as a “combustion bomb”) (c.f. Switsur Reference Switsur1972). This approach is only used for generation of very large gas volumes (2–3 L, e.g., for bulk secondary gases), or specialist radiocarbon analysis of samples (e.g., combustion of large samples to overcome sample inhomogeneity).
Soils and Sediments
If the aim of the radiocarbon measurement of soil or sediment samples (bulk or physical sub-fractions) is to provide chronological information, the standard pretreatment follows the ABA methodology above to remove carbonates and mobile organic carbon fractions. For peat samples, the usual dating target is the base-insoluble (humin) fraction, which remains in solid form after the base step of the ABA treatment. If the base-soluble (humic) fraction of a sample is required, then the solution produced during the base washing of the sample is retained and filtered to remove solid material. This solution containing the dissolved humic fraction is acidified with HCl to precipitate humic material as a solid, which is then washed with MilliQTM water and freeze-dried for analysis.
Where the 14C measurements are to assess the cycling, sources, or storage of carbon in a component of the environmental system, it is important that mobile carbon fractions are retained. Treatment by conventional acid digestion, as described in the preceding sections, would result in loss of some of these fractions. In this case, if the sediment or soil samples contain carbonates, they are pretreated by acid fumigation to remove carbonates before analysis of the organic carbon. This is achieved by placing the sample into a 20 mL glass vial followed by moistening with MilliQTM water. The vial is covered with a filter paper to prevent contamination and placed into a glass vessel together with a beaker of concentrated hydrochloric acid to hydrolyze any carbonate. The fumigation proceeds for 3 days, and the complete removal of carbonate is confirmed by testing. This involves placing a small aliquot of the sample into a gas-tight exetainer vial, which is then flushed with high purity nitrogen gas (Research grade, BOC, UK) before 0.4 mL of 2 M HCl is injected through the exetainer septum. The presence of any remaining carbonate is assessed by measuring any CO2 evolved after the acid injection using a non-dispersive infrared gas sensor [SprintIR, Gas Sensing Solutions, UK].
Following pretreatment, sample CO2 is generated using one of the three approaches described above.
Carbonates
Carbonate samples such as shells, where a sequence of annual or sub-annual growth rings are present, are sub-sampled to isolate the most recent growth phase, provided the aim is measurement of the date of death of the organism. The outer surface of larger samples is visually inspected to identify any evidence of recrystallisation, and mechanically cleaned by abrasion if required. Samples such as corals, speleothems, or travertines are usually supplied with the requirement that all sample material is analysed (rather than sub-sampling). In this case homogenisation proceeds either in the submitter’s laboratory or at NRCL.
Carbonate samples are processed in gas-tight ExetainerTM vials. Usually, this involves pretreating by etching with a calculated volume of 0.2 M HCl to remove the outer 20% by weight of the sample surface. The CO2 evolved from the outer 20% of the sample is then disposed of by flushing with nitrogen gas (Research grade, BOC, UK). Following etching, the remaining sample is completely hydrolyzed to CO2 using an excess of 2 M HCl. The evolved sample CO2 is drawn directly into a vacuum line for cryogenic purification as described below.
Carbon in Waters
Waters are analysed at the laboratory for dissolved organic carbon (DOC), particulate organic carbon (POC) and dissolved inorganic carbon (DIC). For DOC and POC, NRCL recommends collection of sample water in acid-washed bottles (e.g., Gulliver et al. Reference Gulliver, Waldron, Scott and Bryant2010). However, since the laboratory cannot accept samples preserved using mercuric chloride (due to UK government regulations), foil bags which allow the samples to be frozen, can be used when long-term storage of samples prior to analysis is required (c.f. Bryant et al. Reference Bryant, Henley, Murray, Ganeshram and Shanks2013).
POC is isolated from liquid water samples by filtration using ashed (450ºC) glass fiber filters (Whatman GFF). DOC is recovered as solids from filtered water samples by freeze-drying. Sample sizes are typically 0.5–2 L, but the laboratory handles a wide range of sample sizes. In both cases, samples are carefully weighed in order to accurately calculate the carbon concentration in the original sample volume. For both POC and DOC, if decarbonation is required this is achieved by acid fumigation, as described above. The solid, decarbonated, dry sample is then converted to CO2 by combustion, most commonly using the quartz tube method.
Dissolved inorganic carbon is extracted from water samples by acid hydrolysis. Samples of water for analysis are drawn into a gas-tight syringe (150 or 300 mL) fitted with a Quick coupling (Colder Products Co, USA), and 10 mL of 2 M HCl added. The syringe is then attached to a vacuum rig via the coupling and high purity nitrogen gas added to create a headspace in the syringe. The syringe is gently shaken to equilibrate the evolved CO2 into the headspace, and the sample CO2 is recovered by pumping the headspace gases through cryogenic traps as described below.
Carbon in Gases
The laboratory undertakes radiocarbon analysis of the gases carbon dioxide (CO2) and methane (CH4) and has developed a range of sampling methodologies to meet the particular requirements of specific research projects and user community. The CO2-adsorbing properties of zeolite molecular sieve is used in traps for the collection of CO2 samples, which provides a convenient way to collect samples at low CO2 concentrations (e.g., for atmospheric or soil respiration studies; Garnett et al. Reference Garnett, Newton and Ascough2019a). Sample CO2 can be trapped on the molecular sieve by pumping the source air through the zeolite cartridge, and the lab provides portable sampling equipment to facilitate this (Garnett et al. Reference Garnett, Newton and Parker2021), which due to high portability and low power requirements is particularly suitable for fieldwork in remote locations. The same traps can be used as passive samplers, collecting time-integrated samples of CO2 from atmosphere (Garnett and Hartley Reference Garnett and Hartley2010), soil respiration (Garnett et al. Reference Garnett, Hartley, Hopkins, Sommerkorn and Wookey2009) and carbon dioxide dissolved in water (via a hydrophobic filter; Garnett et al. Reference Garnett, Dinsmore and Billett2012). Sample CO2 is recovered from the molecular sieve by heating (425ºC) and purging with high purity nitrogen, followed by cryogenic trapping. The lab also accepts CO2 samples submitted in foil gas sample bags or metal/glass canisters.
Methane samples are submitted to the lab in either foil gas bags, glass flasks or metal canisters, with the latter two being recommended when sample storage prior to analysis is likely to exceed more than several weeks (Garnett et al. Reference Garnett, Murray, Gulliver and Ascough2019b). Methodologies for the collection of CH4 (and CO2) emitted from the surface of peatlands (Garnett et al. Reference Garnett, Hardie and Murray2011) and extracted from freshwaters have been developed (Garnett et al. Reference Garnett, Gulliver and Billett2016; Dean et al. Reference Dean, Billett, Murray and Garnett2017), for which the lab can provide the necessary sampling equipment and training. Methane samples are processed by first removing any traces of CO2 using soda lime and zeolite molecular sieve, before combusting the methane on a catalyst (Platinum-alumina beads, Johnson Mathey Chemicals, UK) heated to 950ºC, followed by cryogenic trapping of the methane-derived CO2 (Garnett et al. Reference Garnett, Murray, Gulliver and Ascough2019b).
Compound Classes and Thermal Fractions. At present the laboratory operates two procedures to isolate specific compound classes of interest from mixed sample matrices. Both methods work on a chemically-defined (as opposed to operationally-defined) basis. For samples where the polyaromatic fraction is of particular interest, hydropyrolysis (HyPy) is used to separate polyaromatic carbon from non-aromatic carbon forms in a sample. The polyaromatic fraction of samples containing many carbon compounds (such as soils, sediments, DOC, Aerosols, etc.) usually relates to “Pyrogenic Carbon” (PyC), which results from the thermal alteration of biomass carbon during fire events (Bird and Ascough, Reference Bird and Ascough2012). This separation is not possible to achieve with many other methods, for example, the final residue after ABA treatment will contain both polyaromatic and non-aromatic material (e.g., cellulose). The HyPy method and instrumentation is described in detail in Ascough et al. (Reference Ascough, Bird, Brock, Higham, Meredith, Snape and Vane2009, Reference Ascough, Bird, Meredith, Wood, Snape, Brock, Higham, Large and Apperley2010), and Meredith et al. (Reference Meredith, Ascough, Bird, Large, Snape, Sun and Tilston2012). Pretreatment of samples by this method involves removal of carbonates via liquid HCl digestion or acid fumigation as described above, followed by addition of the HyPy catalyst [(NH4)2MoO2S2] to the samples. For the majority of samples this is achieved using a mixture of 20 % methanol in MilliQTM water, and precipitating the catalyst onto sample surfaces, but for specific samples (e.g., atmospheric particulates on filters or DOC), the catalyst is added in dry form. Samples are then loaded directly into quartz crucibles for HyPy treatment. The quartz crucibles are produced in-house at SUERC and have the advantage that after HyPy the sample can be combusted in its entirety by the quartz tube method described above, without the requirement for any further sample handling that might result in losses or contamination. Samples are held within the crucibles by precleaned quartz filter paper punches and a gas-permeable quartz frit. The HyPy process uses hydrogen gas (standard grade, BOC, UK) at high temperature (550ºC) and pressure (150 bar) to pyrolytically break the bonds of non-polyaromatic carbon structures within the sample, which are then removed from the HyPy crucible by a H2 sweep gas flow of 5 L per minute. The non-polyaromatic fraction is retained on dry-ice cooled silica for further analysis, if required. Following HyPy, samples are converted to CO2 for 14C measurement using the quartz tube method described above.
For samples containing a range of different carbon compounds of potentially varied radiocarbon content, where any of these carbon compounds is potentially of interest, we have recently developed instrumentation that is based on the approach of Rosenheim et al. (Reference Rosenheim, Day, Domack, Schrum, Benthien and Hayes2008) to liberate different carbon compounds from mixed matrices (e.g., sediments, soils, etc.) on the basis of their thermal stability. This equipment applies ramped oxidation, progressively increasing temperature to evolve sample CO2, which can then be collected over any temperature window during the process for analysis. The evolved sample CO2 is then introduced to a vacuum line for cryogenic purification as described below.
CO2 Purification, Graphitization, and AMS Measurement
Sample CO2 that has been generated by any of the methods described above requires cryogenic purification before aliquots can be taken for stable isotope measurement, graphitization, or archiving. This is achieved on a vacuum rig equipped with spiral cold traps to sequentially remove water and non-condensable gases. Sample CO2 that has been produced by elemental analyser combustion, acid hydrolysis, bomb combustion, molecular sieve desorption, or methane combustion, is fed directly into the vacuum rig for purification. Sample gas that has been produced by the quartz tube combustion method is introduced to the rig by cracking the quartz tube under vacuum in a steel tube cracker.
Sample gases are passed through a “slush” trap made of solid CO2 (dry-ice) and Industrial Methylated Spirits with a temperature of –78ºC. The slush trap isolates water, while sample CO2 is sequentially frozen in two subsequent traps, each of which is surrounded by a Dewar filled with liquid nitrogen (–196ºC). While the sample CO2 is frozen, non-condensable gases are repeatedly pumped away as the sample is moved through the vacuum rig to a cold finger trap, where the volume of CO2 is measured manometrically. From here aliquots of CO2 are taken for off-line δ13C measurement, graphitisation, and any remaining gas is stored as an archive.
The majority of δ13C values for normalisation of sample 14C/13C (or 14C/12C) ratios after AMS measurement are measured on an aliquot of sample CO2 prepared as described above, using isotope ratio mass spectrometry. An aliquot of the sample CO2 that is ≥0.1 mL is measured on a ThermoFisher Delta V stable isotope ratio mass spectrometer with a 10-port multiport. Carbon isotope ratios are reported using the delta (δ) notation (McKinney et al. Reference McKinney, McCrea, Epstein, Allen and Urey1950) with measured values expressed in per mil (‰), where δ is [(Rsample/Rstandard) – 1] x 1000 and R is the ratio of heavy to light isotope (i.e. 13C/12C). Every 9 samples an inhouse gas is used to monitor precision and routinely three international reference materials (IAEA-C1, USGS-24 and IAEA-C5) are also used to ensure accuracy and correct for drift. The reported uncertainty on sample δ13C values as determined from known international standards is less than ± 0.5‰.
Sample CO2 is reduced to solid graphite following the Fe: Zn reduction method of Slota et al. (Reference Slota, Jull, Linick and Toolin1987). Separate quartz aliquot tubes containing zinc (zinc metal powder, Fisher Scientific, UK) and iron (powder, –200 mesh, 99+%, Alfa Aesar, UK) are attached to a single graphitization unit, to which a steel cracking unit containing a glass aliquot tube of sample CO2 is also attached. The sample CO2 is released into the unit by cracking the tube. The quartz tubes containing Fe and Zn are surrounded by furnaces, and the internal furnace temperatures are held within 5ºC of 625ºC (Fe) and 425ºC (Zn). The sample CO2 is reduced to CO over the Zn and is then reduced to C on the surfaces of the Fe powder. The reaction proceeds overnight, during which time the graphite yield is measured manometrically. To ensure that the sample graphite has not been isotopically fractionated a cut-off of 95% graphite yield is applied, above which the sample is acceptable for AMS measurement. When the reaction is complete, the Fe powder on which sample C has precipitated is pressed into an aluminium cathode (National Electrostatics Corporation, USA) for radiocarbon measurement by AMS.
Samples generating >500 µg C are measured on either the SUERC National Electrostatics Corporation (NEC) 5 MV tandem accelerator mass spectrometer (Freeman et al. Reference Freeman, Bishop, Bryant, Cook, Fallick, Harkness, Metcalfe, Scott, Scott and Summerfield2004) or the SUERC NEC 250 kV single-stage accelerator mass spectrometer (SSAMS; Freeman et al. Reference Freeman, Cook, Dougans, Naysmith, Wilcken and Xu2010). Both of these instruments are equipped with 134-position, SNICS (Source of Negative Ions by Caesium Sputtering) ion sources. Samples are measured in up to 13 “groups,” consisting of a mixture of samples and standards. Each “group” contains a primary AMS reference standard and 1 or 2 secondary bulk AMS standards from the international radiocarbon intercomparison programme. In addition, at least three cathodes of 14C-dead (blank) graphite are included to monitor the overall laboratory blank that is associated with aliquoting and graphitisation. The AMS measurement proceeds as described in Dunbar et al. (Reference Dunbar, Cook, Naysmith, Tripney and Xu2016). Samples generating <500 µg C are measured on the Keck Carbon Cycle Accelerator Mass Spectrometry Facility at the University of California, Irvine, USA (KCCAMS/UCI; Southon et al. Reference Southon, Santos, Druffel-Rodriguez, Druffel, Trumbore, Xu, Griffin, Ali and Mazon2004).
Quality Assurance and Data Handling
Oxalic acid II (OXII, National Institute of Standards and Technology, USA) is used as the primary standard for normalisation of measured AMS 14C/12C ratios. Secondary AMS standards are TIRI Barleymash (bulk gas: BBM; Gulliksen and Scott Reference Gulliksen and Scott1995) and Belfast Cellulose (bulk gas: BBC; Boaretto et al. Reference Boaretto, Bryant, Carmi, Cook, Gulliksen, Harkness, Heinemeier, McClure, McGee, Naysmith, Possnert, Scott, van der Plicht and van Strydonck2002). The AMS blank is usually derived from Iceland Spar Calcite (bulk gas: BISC) but an alternative can be generated from a cylinder of ultra-pure 14C-dead CO2 (Air Products, UK), known as APUP2 (see Table 2). Graphite from all of these materials is prepared for each AMS wheel from aliquots of bulk gases. For OXII, BBM and BBC, the bulk gases are produced from large-scale (2–3 L) combustions of solid material on our “combustion bomb” rig (described above). For BISC a large-scale hydrolysis is used, and for APUP2 gas is transferred from the cylinder using a nitrogen-cleaned foil gas bag.
Measured sample 14C/12C or 14C/13C ratios are corrected for isotopic fractionation by one of two methods. Samples measured at the SUERC AMS are corrected using a δ13C value obtained by measurement of an aliquot of the sample CO2 by IRMS (off-line method). For samples measured at the KCCAMS/UCI, fractionation correction is performed using δ13C values obtained in the AMS during measurement of the sample graphite (on-line method), and background correction (and correction for modern carbon contamination, where applicable) is performed as described in Santos et al. (Reference Santos, Southon, Griffin, Beaupre and Druffel2007). Materials for calculation of size-dependant dead and modern carbon contamination are processed alongside the samples and bracket the sample size range. These materials are chosen to match the sample matrix as closely as practical, to behave chemically similarly to the standard materials during all stages of samples processing. For samples measured at the SUERC AMS Laboratory (>500 µg C), a process-specific background is subtracted from the measured sample 14C/13C ratios, after correction for isotopic fractionation (c.f. Donahue et al. Reference Donahue, Jull and Toolin1990). The value and uncertainty for the background correction is obtained from long-term monitoring of 14C-dead standard materials, which have been processed using the method in question.
During laboratory processing of samples, each batch of ≤ 10 samples processed by the NEIF laboratory will typically be accompanied by at least one “process standard.” This will be a 14C-dead material or a known-age material (Table 2). All material types are either international or in-house standards of known homogeneity. In-house standard material has been verified by repeated measurement relative to a verified international 14C standard material (e.g., NIST, IAEA, or International 14C intercomparison material). Measurement of these standards serves two purposes: firstly, a quality assurance (QA) test on every batch of samples processed in the laboratory. The mean and uncertainty of background process standards in an AMS wheel (i.e., material processed at the same time as the samples) is statistically compared with the equivalent background values for long-term monitoring of the same process in the laboratory. This enables the NRCL to check whether any project-specific adjustment is required to the background correction for any particular batch of samples that is processed. The results for known-age process standards are compared to the consensus value via analysis of Z-scores (c.f. Scott et al. Reference Scott, Cook and Naysmith2010). This enables us to confirm the validity of the reported measurement uncertainties and confirm that there is no additional process-specific variability. The other purpose served by process standards is to provide a continuous addition to the long-term running mean of the internal lab QA and appropriate background corrections for any one of the processing methods used by the laboratory that is described above.
The laboratory operates a QA verification program in addition to the routine QA described above. This provides an additional check on the accuracy and precision of background corrections and measurement uncertainties that are applied in reporting of our data. As an example, Figures 1–3 show the results of this QA monitoring program for i) quartz tube combustion, and ii) “routine” ABA processing as described above. In this instance, the monitoring for the QT method covers all stages from preparation of a sample for combustion to final graphitisation, and the monitoring for the ABA method covers all stages from pretreatment of a sample by routine ABA to graphitisation (including quartz tube combustion). These standard materials were processed in the lab and measured at the SUERC AMS facility over the interval between May 2022 to November 2022 inclusive.

Figure 1 QA monitoring of Anthracite background process standards (see Table 2). White circles (Anthracite no p/t) refer to material without pretreatment that was converted to CO2 using the quartz tube combustion method. Gray circles (Anthracite ABA) refer to material treated using the routine ABA method and converted to CO2 using the quartz tube combustion method. Data are given with ±1 σ uncertainties. The solid line represents the average of long-term laboratory Anthracite blank monitoring and dashed lines the 1 σ confidence intervals.
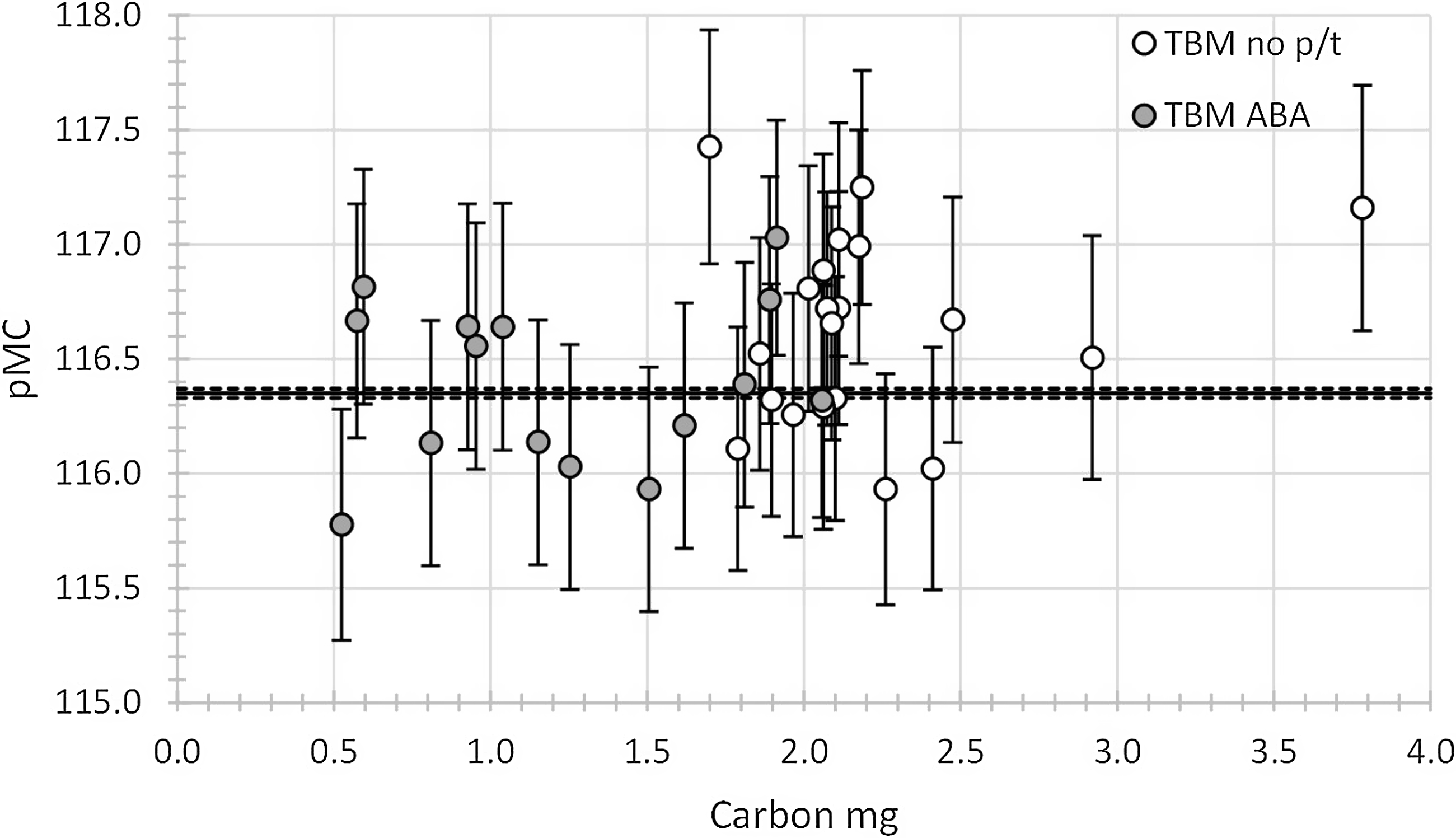
Figure 2 QA monitoring of TIRI Barley Mash (TBM) known-age process standards (see Table 2). White circles (TBM no p/t) refer to material without pretreatment that was converted to CO2 using the quartz tube combustion method. Gray circles (TBM ABA) refer to material treated using the routine ABA method and converted to CO2 using the quartz tube combustion method. Data are given with ±1 σ uncertainties. The solid line represents the TBM consensus value and dashed lines the 2 σ confidence intervals.
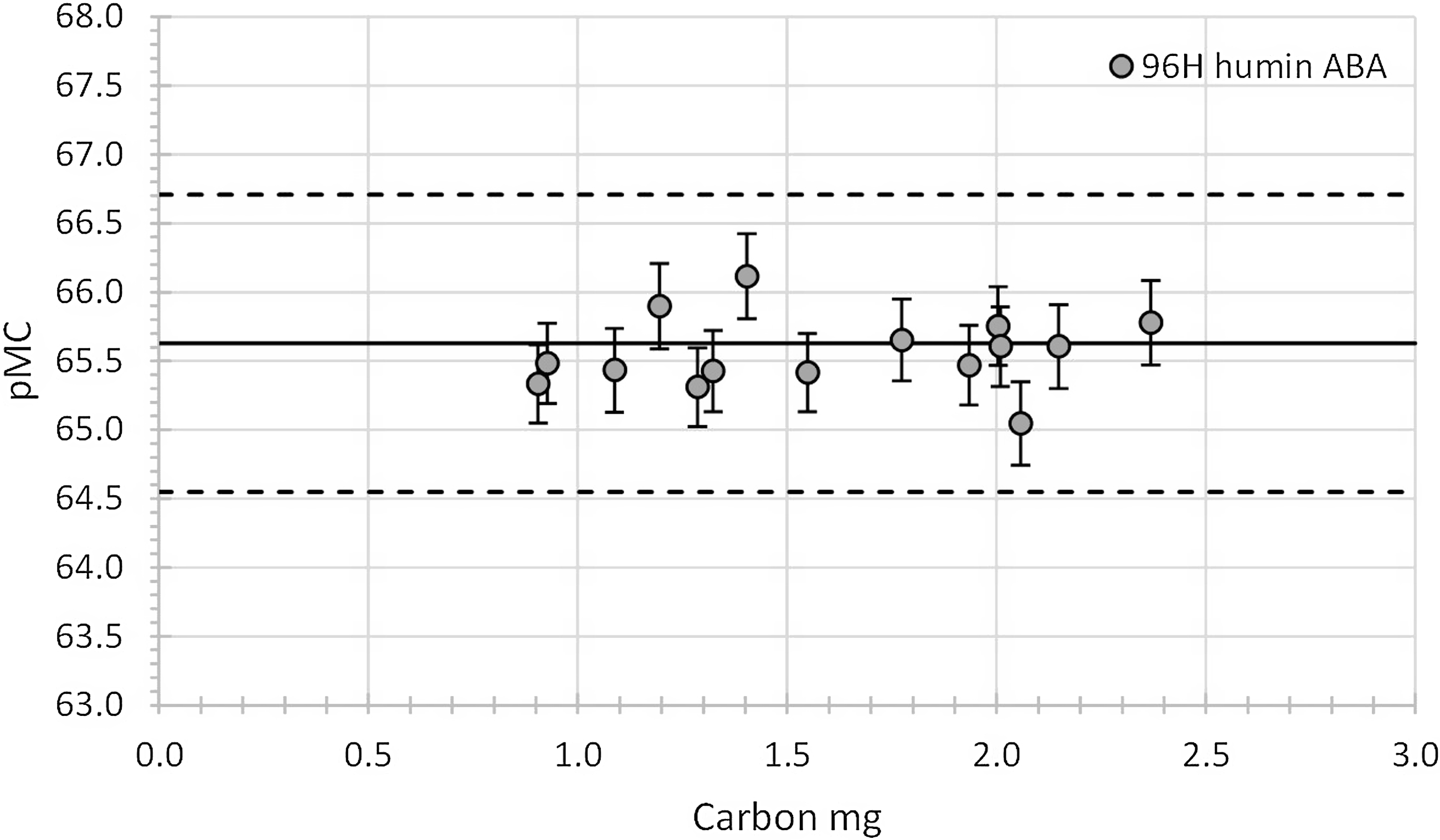
Figure 3 QA monitoring of 96H humin known-age process standards (see Table 2). Gray circles (96H humin ABA) refer to material treated using the routine ABA method and converted to CO2 using the quartz tube combustion method. Data are given with ±1 σ uncertainties. The solid line represents the 96H humin consensus value and dashed lines the 2 σ confidence intervals.
ACKNOWLEDGMENTS
We acknowledge support from NERC via the National Environmental Isotope Facility (NEIF) grant funding (NE/S011587/1). We gratefully acknowledge the staff of the SUERC AMS laboratory and the Keck Carbon Cycle Accelerator Mass Spectrometry Facility, University of California, Irvine, USA (KCCAMS/UCI) for support with AMS measurements of graphite. At the KCCAMS/UCI we particularly acknowledge the assistance of Xiaomei Xu regarding the analysis of graphite targets <500 µg C. We also gratefully acknowledge the staff of the Scottish Universities Environmental Research Centre for support, discussions, and encouragement.
COMPETING INTERESTS
The authors declare no competing interests relating to this manuscript.