Chronic consumption of a diet containing high fat and high sucrose is known to cause obesity. Obesity, the cause of metabolic disorders, such as hyperglycaemia, insulin resistance and type 2 diabetes(Reference Kao, Chang and Lee1–Reference Ding, Li and Song3), also affects gut hormone secretion(Reference Sakar, Duca and Langelier4). Glucose homeostasis is regulated by various hormones, such as glucagon, insulin, glucocorticoids and incretins (glucose-dependent insulinotropic polypeptide and glucagon-like peptide-1 (GLP-1)). GLP-1, produced in enteroendocrine L-cells, is released in response to ingested nutrients, such as glucose, fatty acids, proteins and amino acids(Reference De León, Li and Delson5–Reference Hira, Ikee and Kishimoto10). Due to its incretin effect (stimulating insulin secretion), increased GLP-1 secretion plays a role in normalising the postprandial glucose homeostasis(Reference Cho, Fujita and Kieffer11). Incretin-based therapy is effectively used for type 2 diabetes treatment. Therefore, increasing GLP-1 secretion may be a promising target for prevention and treatment of glucose intolerance(Reference Nauck12).
Up to date, a consensus on how does GLP-1 secretion/production change during the progression of diet-induced obesity (DIO) has not been established(Reference Nauck, Vardarli and Deacon13,Reference Nauck and Meier14) . Studies report that GLP-1 secretion is diminished during obesity development(Reference Gniuli, Calcagno and Dalla Libera15–Reference Toft-Nielsen, Damholt and Madsbad17). In contrast, we previously demonstrated that GLP-1 secretion in response to normal diet administration was increased by chronic consumption of high fat and high sucrose in rats, at least in the early stage of DIO(Reference Nakajima, Hira and Hara18). Thus, the role of endogenous GLP-1 in the development of glucose intolerance and obesity has not been clearly identified.
The present study aims to clarify the role of endogenous GLP-1 in postprandial glycaemia during progression of DIO. For this, a GLP-1 receptor antagonist, exendin (9–39) (Ex9), was chronically delivered using an osmotic pump implanted subcutaneously in rats fed an obesogenic high-fat and high-sucrose diet. Ex9, a thirty-one-amino-acid peptide, is a specific and competitive antagonist of the GLP-1 receptor and is widely used to block GLP-1 signalling(Reference Edwards, Todd and Mahmoudi19–Reference Coopman, Wallis and Robb22). Meal tolerance tests (MTT) using a liquid diet rather than the conventional glucose solution were conducted to assess postprandial responses of glucose, insulin and GLP-1. Simultaneously, the gastric emptying rate was evaluated during the MTT.
Material and methods
Animals and diets
Male Sprague–Dawley rats (5 weeks old, weight about 120–150 g) were purchased from Japan SLC, Inc. and given an American Institute of Nutrition-93G (control) diet(Reference Reeves23) for 1 week to adapt to their new environment before conducting the experiment. Rats were housed individually and maintained in a temperature-controlled room (22 ± 2°C) at 50 ± 5 % humidity with a standard light cycle (08.00–20.00 hours light period). All rats were given access to diet and water ad libitum. The GLP-1 receptor antagonist, Ex9 (analytical grade, purity > 95 %), was purchased from Thermo Fisher Scientific Inc. Ex9 was dissolved in sterile saline and transferred to the Alzet Mini osmotic pump (Model 2006, Durect corporation) following the manufacturer’s instructions. According to a previous study, Ex9 impairs glucose tolerance during an oral glucose tolerance test (OGTT)(Reference Dusaulcy, Handgraaf and Skarupelova24). In our study, 100 µg/day per rat of Ex9 was used to block GLP-1 signalling. Rats were anaesthetised with sodium pentobarbital (50 mg/kg body weight, Somnopentyl injection; Kyoritsu Seiyaku Corporation) and subcutaneously implanted with the osmotic pump in the interscapular region. Ex9 or vehicle was delivered at a constant rate of 0·13 µl/h throughout the experimental period (35 d).
Rats were divided into three groups (n 8–9/group) to have comparable body weight, glucose and GLP-1 levels based on measurements the day prior to starting test diet feeding and Ex9 administration. The control group was fed a control diet with continuous saline administration, the high-fat/high-sucrose (HFS) group was fed a HFS diet (30 % fat and 40 % sucrose, shown in Table 1)(Reference Nakajima, Hira and Hara18,Reference Hira, Suto and Kishimoto25) with continuous saline administration and the Ex9 group was also fed the HFS diet with continuous Ex9 administration for 5 weeks. Body weight and food intake were measured every 2 d. All experimental animal procedures were approved by the Hokkaido University Animal Committee, and animals were maintained in accordance with the Hokkaido University guidelines for the care and use of laboratory animals. Because we aimed to clarify the role of enhanced GLP-1 response in DIO model rats, and because the role of GLP-1 in insulin secretion is well established in normal conditions, we examined the effect of Ex9 in the HFS-fed rats, not in the control-fed rats.
Table 1. Experimental diet composition (g/kg of diet)

HFS, high-fat/high-sucrose.
* Acid casein (Fonterra, Ltd.).
† TK-16 (Matsutani Chemical Industry Co., Ltd.).
‡ Just Fiber BH200FCC (Morimura Bros., Inc.).
§ Mineral and vitamin mixtures were prepared according to the American Institute of Nutrition 93G formulation.
Meal tolerance tests
Following the feeding periods of 2 and 4 weeks, MTT were conducted to determine postprandial glucose, insulin, GLP-1 and gastric emptying responses to ‘meal’ administration. Rats were fasted for 16 h before basal blood collection (0 min) and were then orally administered a liquid diet (15 kcal (63 kJ)/10 ml per kg body weight; Ensure H, Abbott) premixed with acetaminophen (100 mg/kg body weight; Sigma Aldrich). Blood samples were collected from the tail vein at 15, 30, 60, 90 and 120 min following the liquid diet administration and immediately transferred to chilled tubes containing heparin (final concentration at 50 IU/ml blood; Ajinomoto Company, Inc.) and aprotinin (final concentration at 500 Kallikrein inhibitor units (kIU)/ml blood; Wako Pure Chemical Industries, Ltd.). Blood samples were centrifuged at 2300 g for 10 min at 4°C and plasma samples were collected and stored at −80°C until further analysis. Glucose levels were measured using the Glucose CII Test Kit (Wako Pure Chemical Industries). Gastric emptying rate was assessed by the acetaminophen (paracetamol) absorption test(Reference Maida, Lovshin and Baggio26–Reference Medhus, Lofthus and Bredesen28). Acetaminophen levels were determined using an acetaminophen detection kit (Kanto Chemical Co., Inc.). Plasma insulin was measured using an insulin ELISA (U-E type, AKRIN-130; Shibayagi Company Limited). The Rat Insulin ELISA assay has a range of 0·156–10 ng/ml. Antibodies in this kit are specific to insulin which cross-reacts with rat proinsulin, mouse insulin and human insulin. The intra-assay precision and inter-assay precision are < 2 % and < 3 %, respectively. GLP-1 concentration was determined using Multi Species GLP-1 Total ELISA Kit (EZGLP1T-36K; Merck Millipore). The GLP-1 Total ELISA detects both GLP-1 (7–36) and GLP-1 (9–36) and has no significant cross-reactivity with GLP-2, GIP, glucagon and oxyntomodulin. The minimum detection limit of the assay is 1·5 pM. The intra-assay precision and inter-assay precision are < 5 % and < 12 %, respectively.
Blood and tissue collection
Following a 5-week feeding period and an overnight fasting, blood samples were taken from the portal vein and abdominal aorta of rats under sodium pentobarbital anaesthesia (50 mg/kg of body weight) using a syringe filled with heparin (final concentration 50 IU/ml), aprotinin (final concentration 500 KIU/ml) and dipeptidyl peptidase (DPP)-IV inhibitor (final concentration 50 µmol/l; DPP4-010; Merck Millipore. Plasma was collected and stored as described above for measurements of glucose, insulin, total GLP-1, TAG and total cholesterol levels. For measurement of DPP-IV activity, blood samples were simultaneously collected from the portal vein and abdominal aorta into a syringe containing only heparin (final concentration 50 IU/ml). Total GLP-1 and insulin levels were assessed using Multi Species GLP-1 Total ELISA (EZGLP1T-36K; Merck Millipore) and Rat Insulin ELISA (AKRIN-010T; Shibayagi Company Limited) kits, respectively. Plasma TAG and cholesterol levels were measured using the TAG E-Test and Cholesterol E-Test kits (Wako Pure Chemical Industries), respectively. DPP-IV activity was measured based on the rate of surrogate substrate (Gly-Pro-p-nitroaniline; Gly-Pro-pNA) hydrolysis as described previously(Reference Karl, Chwalisz and Wedekind29–Reference Ishikawa, Hira and Inoue31).
Rats were killed by exsanguination and intestinal segments were immediately dissected and washed with a cold saline solution (0·9 % NaCl). Sections (2 cm) of the jejunum, ileum and colon were taken from the middle region of each dissected segment. Caecum tissues were washed with cold saline and divided equally into two parts, and 2 cm from the middle region was collected. All intestinal samples for GLP-1 measurement were immediately frozen in liquid N2 and stored at −80°C until further analysis. Mesenteric, retroperitoneal and epididymal adipose tissues were carefully dissected and weighed to determine the visceral adipose tissue weight.
Measurement of glucagon-like peptide-1 content in intestinal tissue
An ethanol–acid solution was prepared by mixing absolute ethanol, water and 12 M HCl at a ratio of 74:25:1(Reference Cani, Hoste and Guiot32). Tissue samples were submerged in the ethanol acid solution (5 ml/g tissue) and homogenised at 25 000 rpm (Ultra-Turrax homogeniser T18, IKA) for 2 min. Homogenised samples were placed at 4°C for 24 h. After 24 h, aliquots (150 µl) of homogenate were stored separately at −30°C until protein analysis. The remaining homogenate was centrifuged at 2000 g for 20 min. The supernatant was collected and stored at −80°C until analysed. Protein and GLP-1 content were measured using Lowry’s protein assay and Multi Species GLP-1 Total ELISA kit (EZGLP1T-36K; Merck Millipore), respectively. Homogenate samples were diluted 30-fold for protein measurement, whereas the supernatant was diluted 500- or 1000-fold (colon only) for GLP-1 measurement.
Statistical analysis
Sample size was calculated based on the experimental design (two-way repeated-measures ANOVA) for MTT to determine the role of endogenous GLP-1 in glucose homeostasis as the primary outcome measure using G*Power software (version 3.1.9.2) following the power = 0·8, significant level = 0·05 and effect size = 0·25. According to Cohen’s effect size conventions for ANOVA F tests(Reference Cohen33), we used medium effect size for the total sample size calculation(Reference Bot, Pouwer and Assies34,Reference Qin, Sun and Huang35) . Hence, the total number of rats was calculated as twenty-four (eight rats/group) in the present study. Results are presented as means with their standard errors. Significant effects of time, treatment and the interactions of time and treatment were assessed by two-way repeated ANOVA in the MTT results. One-way ANOVA, Tukey–Kramer’s test or Student’s t test were used to determine significant differences among treatment groups with P < 0·05 considered statistically significant. Data were statistically analysed using JMP pro version 13.0 software (SAS Institute, Inc.).
Results
Body weight, energy intake and tissue weights
There were no significant differences in the initial body weights of the rats between groups (182·1 (se 2·5) to 184·0 (se 2·7) g, P = 0·9000). At the end of the 5-week feeding period, average body weights of the HFS (P = 0·0054) and Ex9 (P = 0·0209) groups were significantly higher than the control group (Fig. 1(A)). HFS and Ex9 groups had higher body weight gain (P = 0·0008 and P = 0·0030), energy intake (P = 0·0011 and P = 0·0073), visceral adipose tissue weights (P = 0·0005 and P = 0·0069) (mesenteric (P = 0·0091 and P = 0·0473), epididymal (P = 0·0007 and P = 0·0078) and retroperitoneal (P = 0·0002 and P = 0·0030)) and liver weight (P = 0·0003 and P = 0·0007) than the control group (Fig. 1(B)–(H)). No significant differences were observed between the HFS and Ex9 groups in any parameters.

Fig. 1. Body weight, energy intake and tissue weights after a 5-week feeding period of control and high-fat/high-sucrose (HFS) diets with or without continuous exendin (9–39) (Ex9) administration. Rats were provided either a control or HFS diet with addition of either saline or Ex9 administration (100 µg/d) for 5 weeks. Values are means, with standard errors represented by vertical bars (n 8–9 rats in each group). a,b Mean values with unlike letters were significantly different between treatments (P < 0·05, Tukey–Kramer’s test). * To convert kcal to kJ, multiply by 4·184.
Basal glucose, insulin and glucagon-like peptide-1 levels
After the 2- and 4-week feeding periods, rats were fasted overnight (16 h) before conducting the MTT. No significant differences were observed in any of the measured parameters after 2 and 4 weeks (Fig. 2). Basal glucose (P = 0·4547) and GLP-1 (P = 0·1243) levels did not differ between any groups (Fig. 2(D) and (F)), whereas the basal insulin level tended to be higher in the HFS group compared with the control group (P = 0·0587) after 4 weeks (Fig. 2(E)).

Fig. 2. Basal glucose, insulin and glucagon-like peptide-1 (GLP-1) levels after the 2- and 4-week feeding periods of control and high-fat/high-sucrose (HFS) diets with or without continuous exendin (9–39) (Ex9) administration. Rats were provided a control or HFS diet with either saline or Ex9 administration (100 µg/d) for 2 and 4 weeks before conducting meal tolerance tests. On the experiment day, blood samples from the tail vein were collected at 0 min (basal level) after overnight fasting (16 h). Values are means, with standard errors represented by vertical bars (n 8–9 rats in each group). NS indicates no significant difference among the treatments (P < 0·05, Tukey–Kramer’s test). * To convert glucose in mg/dl to mmol/l, multiply by 0·0555.
Postprandial glycaemic, insulin, glucagon-like peptide-1 and gastric emptying responses during meal tolerance tests after a 2-week feeding period
In this experiment, an MTT using a liquid diet was conducted to determine postprandial glucose, insulin, GLP-1 and gastric emptying during obesity development. After a 2-week feeding period, postprandial glucose levels in the Ex9 group were tended to be higher compared with the HFS and control groups from 30 to 60 min. The glucose level at 90 min (P = 0·0079) and AUC (P = 0·0337) in the Ex9 group were significantly higher than the control group (Fig. 3(A) and (B)). The postprandial insulin level at 15 min in the HFS group was significantly higher than the control group (P = 0·0216), whereas the Ex9 group showed an intermediate level between the HFS and control groups (Fig. 3(C)). The AUC of insulin in the HFS group was larger than in the control groups (P = 0·0486) (Fig. 3(D)). Postprandial GLP-1 secretion following liquid diet administration in the HFS (P = 0·3829) and Ex9 (P = 0·5708) groups was slightly higher than in the control group (Fig. 3(E) and (F)). There was a significant effect of treatment (P < 0·05) on the GLP-1 response assessed by two-way repeated-measures ANOVA. Changes in plasma acetaminophen concentrations were not different between treatments (Fig. 3(G)).
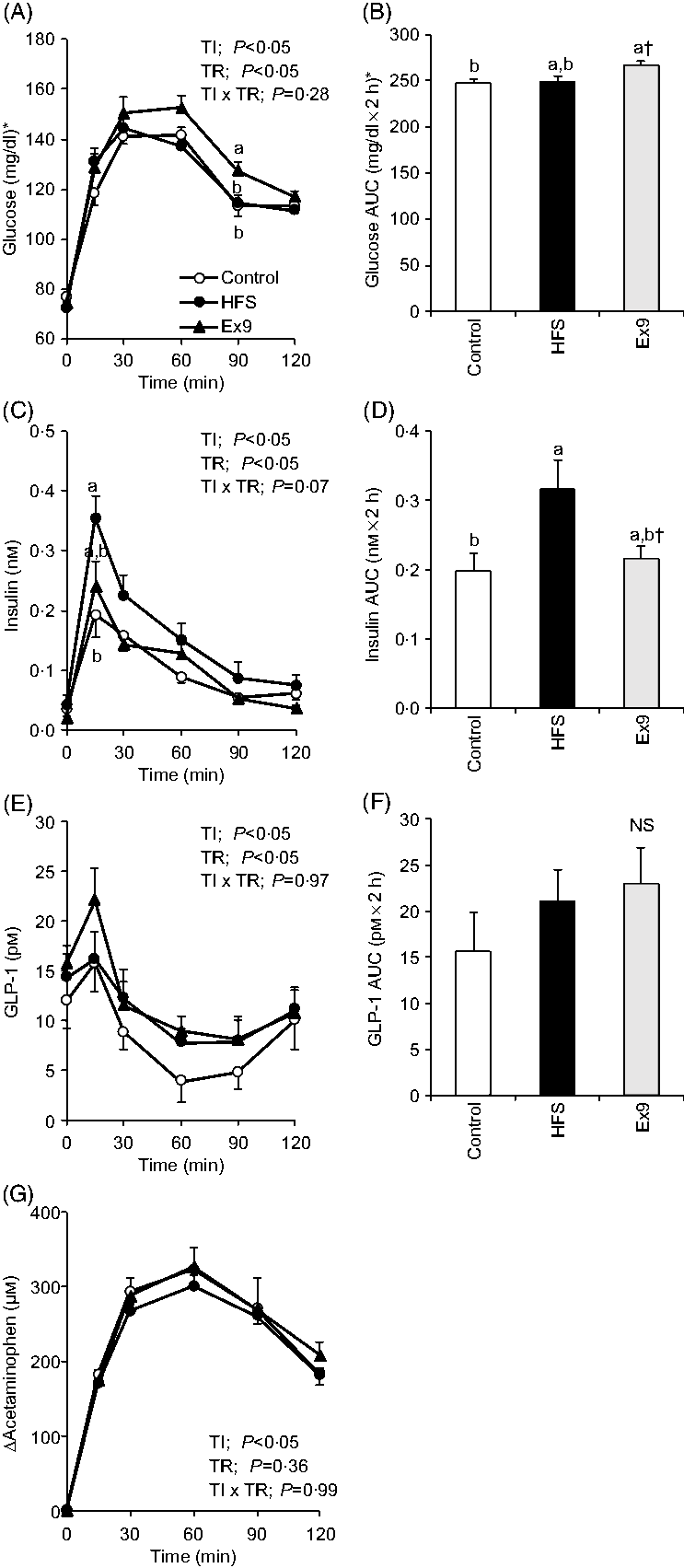
Fig. 3. Postprandial glucose, insulin, glucagon-like peptide-1 (GLP-1) response and gastric emptying rate during meal tolerance tests (MTT) after a 2-week feeding period of control and high-fat/high-sucrose (HFS) diets with or without continuous exendin (9–39) (Ex9) administration. Rats were provided a control or HFS diet with either saline or Ex9 administration (100 µg/d) for 2 weeks before conducting MTT. After an overnight fasting, blood samples were collected from the tail vein before (0 min) and after oral administration of a liquid diet (Ensure H, 10 ml/kg body weight). Values are means, with standard errors represented by vertical bars (n 8–9 rats in each group). a,b Mean values with unlike letters were significantly different between treatments (P < 0·05, Tukey–Kramer’s test). † Significant differences between mean values of the HFS and Ex9 groups (P < 0·05, Student’s t test). NS indicates that there was no significant difference among treatments. TI, time; TR, treatment. * To convert glucose in mg/dl to mmol/l, multiply by 0·0555.
Postprandial glycaemic, insulin, glucagon-like peptide-1and gastric emptying responses during meal tolerance tests after 4-week feeding period
Postprandial glucose levels in the Ex9 group were slightly higher than the control group (15–90 min) and significantly (120 min) (P = 0·0241) higher than the control group following administration of the liquid diet (Fig. 4(A)). The AUC of glucose in the Ex9 group was significantly higher than in the control group (P = 0·0036) (Fig. 4(B)), while the HFS group had a similar glycaemic response compared with the control group (P = 0·3475). In contrast to the glucose response, the HFS group had a higher level of insulin secretion than the other groups (P = 0·0081) (Fig. 4(C) and (D)). Basal GLP-1 levels in the HFS (P = 0·1088) and Ex9 (P = 0·3791) groups were slightly increased compared with that in the control group. But GLP-1 levels at 60 (P = 0·0395) and 90 min (P = 0·0422) in the HFS group and at 90 min in Ex9 group (P = 0·0343) became significantly higher than the control group, demonstrating that the postprandial GLP-1 response was larger in the HFS and Ex9 groups than the control group (Fig. 4(E)). Significant effects of treatment on postprandial glycaemia, insulin and GLP-1 secretion were detected by two-way repeated-measures ANOVA in both MTT (Figs. 3 and 4). Plasma acetaminophen responses at all time points in the HFS and Ex9 groups were similar to the control group (Fig. 4(G)).

Fig. 4. Postprandial glucose, insulin, glucagon-like peptide-1 (GLP-1) responses and gastric emptying rate during meal tolerance tests (MTT) after a 4-week feeding period of control and high-fat/high-sucrose (HFS) diets with or without continuous exendin (9–39) (Ex9) administration. Rats were provided a control or HFS diet with either saline or Ex9 administration (100 µg/d) for 4 weeks before conducting MTT. After an overnight fasting, blood samples were collected from the tail vein before (0 min) and after oral administration of a liquid diet (Ensure H, 10 ml/kg body weight). Values are means, with standard errors represented by vertical bars (n 8–9 rats in each group). a,b Mean values with unlike letters were significantly different between treatments (P < 0·05, Tukey–Kramer’s test). † Significant differences between mean values of the HFS and Ex9 groups (P < 0·05, Student’s t test). TI, time; TR, treatment. * To convert glucose in mg/dl to mmol/l, multiply by 0·0555.
Plasma parameters after 5-week feeding period
Blood samples were collected from the portal vein and abdominal aorta after overnight fasting to measure fasting gut hormone levels and other parameters. Consistent with postprandial GLP-1 secretion at 4 weeks, both the HFS (P = 0·0011) and Ex9 (P = 0·0113) groups showed significantly higher GLP-1 concentrations compared with the control group (Fig. 5(C)). DPP-IV activity in both the portal vein (P = 0·5414) and abdominal aorta (P = 0·3735) was not different among the groups (Figs. 5(D) and 6(E)). Glucose levels were significantly higher in the Ex9 group compared with the HFS and control groups, both in the portal vein (P = 0·0022) and abdominal aorta (P = 0·0252) (Figs. 5(A) and 6(A)). Similar to the postprandial insulin response, portal/aorta insulin levels in the HFS group were higher than in the control group (P = 0·0198 and P = 0·0431), while the Ex9 group had an intermediate value between the HFS and control groups (Figs. 5(B) and 6(B)). TAG and cholesterol levels in the HFS (P = 0·0416 and P = 0·0116) and Ex9 (P = 0·0569 and P = 0·0611) groups were higher than in the control group Fig. 6(C) and (D)).

Fig. 5. Portal glucose, insulin, glucagon-like peptide-1 (GLP-1) levels and dipeptidyl peptidase (DPP)-IV activity after a 5-week feeding period of test diets with or without continuous exendin (9–39) (Ex9) administration. Rats were provided a control or high-fat/high-sucrose (HFS) diet with either saline or Ex9 administration (100 µg/d) for 5 weeks. Blood samples from the portal vein and abdominal aorta were collected under sodium pentobarbital anaesthesia (50 mg/kg body weight) after overnight fasting (16 h). Values are means, with standard errors represented by vertical bars (n 8–9 rats in each group). a,b Mean values with unlike letters were significantly different between treatments (P < 0·05, Tukey–Kramer’s test). NS indicates that there was no significant difference among treatments. * To convert glucose in mg/dl to mmol/l, multiply by 0·0555.

Fig. 6. Aortic glucose, insulin, TAG, cholesterol levels and dipeptidyl peptidase-IV (DPP-IV) activity in abdominal aorta after a 5-week feeding period of test diets with or without continuous exendin (9–39) (Ex9) administration. Rats were provided a control or high-fat/high-sucrose (HFS) diet with either saline or Ex9 administration (100 µg/d) for 5 weeks. Blood samples from the portal vein and abdominal aorta were collected under sodium pentobarbital anaesthesia (50 mg/kg body weight) after overnight fasting (16 h). Values are means, with standard errors represented by vertical bars (n 8–9 rats in each group). a,b Mean values with unlike letters were significantly different between treatments (P < 0·05, Tukey–Kramer’s test). NS indicates that there was no significant difference among treatments. * To convert glucose in mg/dl to mmol/l, multiply by 0·0555; to convert TAG in mg/dl to mmol/l, multiply by 0·0113; to convert cholesterol in mg/dl to mmol/l, multiply by 0·0259.
Glucagon-like peptide-1 content in the intestinal tissues
As expected, GLP-1 content gradually increased along the proximal–distal axis of intestinal regions. In the ileal segment, the GLP-1 content of the HFS group (P = 0·0094) was significantly higher than in the control group (Fig. 7(C)). The Ex9 group (P = 0·1016) also showed a similar tendency, but slightly less than the HFS group. Conversely, there were no significant differences in GLP-1 concentration in the other intestinal segments (Fig. 7).

Fig. 7. Glucagon-like peptide-1 (GLP-1) content in intestinal tissues after a 5-week feeding period of a control or high-fat/high-sucrose (HFS) diet with or without continuous exendin (9–39) (Ex9) administration. Rats were provided a control or HFS diet with either saline or Ex9 administration (100 µg/d) for 5 weeks. Each intestinal segment: (A) duodenum, (B) jejunum, (C) ileum, (D) caecum and (E) colon were collected after killing. Values are means, with standard errors represented by vertical bars (n 8–9 rats in each group). a,b Mean values with unlike letters were significantly different between treatments (P < 0·05, Tukey–Kramer’s test). NS indicates that there was no significant difference among treatments.
Discussion
The primary purpose of the present study was to determine the physiological relevance of increased/decreased postprandial GLP-1 secretion during development of DIO or glucose intolerance. We found that normalisation of the postprandial glycaemic response was accompanied by an increase in insulin and GLP-1 secretion in rats fed an HFS diet, whereas continuous GLP-1 receptor antagonist administration caused glucose intolerance. These results indicate that enhancement of the postprandial GLP-1 response plays an important role in preventing glucose intolerance development during DIO.
In general, a high-fat and/or high-sucrose diet has been used to establish obesity and metabolic disorder animal models(Reference Yang, Miyahara and Takeo2,Reference Long, Zhang and Sun36–Reference Sumiyoshi, Sakanaka and Kimura38) . In this experiment, rats were fed an HFS diet (40 % fat and 30 % sucrose) for 5 weeks in order to monitor chronic changes in postprandial glucose, insulin and GLP-1 responses during progression of DIO. Our results suggest that a diet containing high fat and high sucrose establishes obesity in an animal model, even in a shorter period (5 weeks) than generally employed (8–12 weeks or longer). However, body weight, body weight gain and visceral adipose tissue weight in the HFS and Ex9 groups were similar levels. It can be assumed that longer experimental periods are required to observe the effect of blocking endogenous GLP-1 signal on these parameters.
Postprandial glycaemic and insulin responses are generally assessed using a classical OGTT. In the present study, oral administration of a liquid diet (Ensure H, containing all of nutrients) was employed in MTT as it is more appropriate methodology for investigating postprandial metabolic responses, compared with OGTT(Reference Brodovicz, Girman and Simonis-Bik39). Postprandial GLP-1 levels in the HFS and Ex9 groups were slightly higher after a 2-week feeding and significantly higher after a 4-week feeding than the control group. Our results confirmed that chronic consumption of a high-fat and high-sucrose diet enhances postprandial GLP-1 secretion in response to the meal administration(Reference Nakajima, Hira and Hara18).
In the present study, gastric emptying rates were unchanged by the chronic HFS diet with or without Ex9 compared with the control group; therefore, enhanced GLP-1 responses in the HFS and Ex9 groups cannot be explained by differences in the rate of nutrient load into the small intestine from the stomach. Although Ex9 has been previously reported to accelerate gastric emptying(Reference Edwards, Todd and Mahmoudi19,Reference Deane, Nguyen and Stevens40) , the results suggest the role of endogenous GLP-1 as incretin hormone, independently of effect on gastric emptying(Reference Witte, Grybäck and Jacobsson41). In addition, the gastric emptying rate is regulated by various factors such as ghrelin, serotonin, CCK, PYY, not only on GLP-1(Reference Nakajima, Hira and Hara18,Reference Hellström, Grybäck and Jacobsson42) . In line with our study, gastric emptying was not changed by Ex9 treatment(Reference Witte, Grybäck and Jacobsson41,Reference Nicolaus, Brödl and Linke43) . Increased GLP-1 production in the ileum may contribute to the increased GLP-1 secretion. It is also speculated that the sensitivity of L-cells to luminal nutrients was increased following chronic consumption of a high-fat and high-sucrose diet. No significant differences were observed in GLP-1 concentration in the other intestinal segments, suggesting that L-cells located in the ileum segment are more susceptible to chronic obesogenic diet feeding than other intestinal segments. It could be implied that the ileum might play an important role in postprandial GLP-1 release. This was supported by the findings that postprandial GLP-1 secretion is stimulated by luminal contents on L-cells in the proximal ileum(Reference Singh44,Reference Wang, Liu and Chen45) . In the present study, we did not observe compensatory elevation of GLP-1 secretion by Ex9 treatment, compared with the HFS group. It can be assumed that GLP-1 secretion in both high-fat and high-sucrose feeding groups (HFS and Ex9) might be the maximum level that can be secreted from the L-cells in our experimental models. Compensatory elevations of GLP-1 by Ex9 have not always been found in previous studies(Reference Schirra, Nicolaus and Roggel46,Reference Calabria, Li and Gallagher47) . Although both the HFS and Ex9 groups showed elevated postprandial GLP-1 secretion compared with the control group, postprandial insulin secretion was diminished in the Ex9 group compared with the HFS group. These results clearly demonstrate that postprandial GLP-1 was effectively blocked by Ex9 treatment, resulting in insufficient insulin secretion. Accordingly, our results illustrate that chronic HFS feeding with continuous Ex9 administration rapidly established postprandial hyperglycaemia, while HFS alone maintained a glycaemic response similar to the control group in both the 2- and 4-week feeding periods. Previous studies demonstrated that Ex9 treatment for 14 d accelerated glucose intolerance in rats assessed by OGTT(Reference Dusaulcy, Handgraaf and Skarupelova24) and intraperitoneal injection of Ex9 prior to OGTT-induced glucose intolerance in rats(Reference Tseng, Zhang and Wolfe48). Overnight fasting (14–18 h) is generally employed in typical metabolic studies(Reference Ayala, Samuel and Morton49,Reference Yamazaki, Yasuda and Inoue50) , but prolonged fasting may affect glycaemic response and improve insulin sensitivity, compared with relatively shorter(Reference Ayala, Bracy and McGuinness51–Reference Chen, Zhou and Saha53). However, we previously did not observe impaired glycaemic response after 6-h fasting in DIO rats fed the HFS diet for 4 weeks(Reference Nakajima, Hira and Hara18). That result (6-h fasting) and the present result (overnight fasting) suggest that postprandial glucose tolerance was partially maintained in rats fed the HFS diet, regardless of fasting periods. It can be concluded that elevation of postprandial GLP-1 plays an important role in the prevention and amelioration of glucose intolerance during DIO through increasing insulin responses. Hence, enhancing endogenous GLP-1 secretion could be a potential target for prevention of glucose intolerance and obesity development.
After a 5-week feeding period, the fasting glucose level in the HFS group was similar to the level of the control group, whereas fasting GLP-1 and insulin levels were elevated in the HFS group. In the Ex9 group, the fasting glucose level was higher with a partially lower insulin level compared with the HFS group. These results demonstrate that not only postprandial GLP-1 but also fasting GLP-1 plays an important role in the prevention of blood glucose elevation. Similar elevations in fasting TAG and cholesterol levels in the HFS and Ex9 groups suggest that endogenous GLP-1 does not directly affect dyslipidaemia under the experimental conditions.
Liquid diet Ensure H contains nutrients such as carbohydrates, proteins, lipids, vitamins and minerals, which are used for nutrient preservation after surgery or as nutritional support. It has been reported that GLP-1 secretion is stimulated by proteins, carbohydrates, TAG and fatty acids(Reference Carr, Larsen and Winzell6,Reference Tolhurst, Reimann and Gribble7,Reference Sakamoto, Seino and Fukami54,Reference Holst55) . In this experiment, the effect of specific diet components that stimulate postprandial GLP-1 secretion still has not been investigated. Thus, examining a single nutrient during an MTT is needed in future studies to determine the adaptive changes of single-nutrient-induced postprandial GLP-1 secretion during development of DIO.
Conclusions
The postprandial glycaemic response in the HFS group was similar to the control group, whereas postprandial GLP-1 and insulin secretion were increased in the HFS group. In the Ex9 group, which blocked the GLP-1 signal, the postprandial glycaemic response was elevated with a lower insulin response compared with the HFS group. It could be concluded that enhancement of postprandial GLP-1 induction by chronic feeding of an obesogenic diet has a role in maintaining normal postprandial glycaemia in diet-induced obese rats.
Acknowledgements
This work was supported by JSPS KAKENHI grant number 16K07725.
J. P., T. H. and H. H. designed the research; J. P. conducted the research and analysed the data; J. P. and T. H. wrote the paper; T. H. had primary responsibility for the final content. All authors read and approved the final manuscript.
The authors declare that they have no conflicts of interest.