Introduction
The Three Gorges Reservoir (TGR) is the world's largest hydropower project and the most important water conservancy regulation scheme. The total water storage capacity and the flood regulation capacity of this reservoir are 39.3 km3 and 22.1 km3, respectively, enabling hydropower generation, disaster reduction, and navigation improvement (Fu et al. Reference Fu, Wu, Lü, Xu, Cao, Niu, Yang and Zhou2010; Zhang and Lou Reference Zhang and Lou2011). The impoundment of the Three Gorges Reservoir caused a great number of environmental problems, however, most of which were related to sediment deposition (Wu et al. Reference Wu, Huang, Han, Xie and Gao2003; Xu et al. Reference Xu, Tan and Yang2013a).
Sediment deposition has affected river processes in navigation channels and ports in backwaters, as well as the smooth operation of power plants and navigation gates. Due to the deposition of sediment in the TGR, the sediment concentration in the water flowing downstream has been greatly reduced, which increased the scouring of the downstream riverbed by the current, resulting in a lowering of the water level and further affecting inland navigation. At the same time, sediments are the main carriers of pollutants such as nutrients and heavy metals, thus promoting the development of eutrophication of the TGR aquatic ecosystem (Xu et al. Reference Xu, Tan and Yang2013a). The sediment accumulation was estimated to be 1.67×109 tons with a mean deposition flux of ~1.11×108 ton/y in the TGR during 2003–2017 according to the Yangtze River Sediment Bulletin (CWRC 2003–2017). At present, dredging sediments is a popular way to inhibit the rapid increase in sediment deposition in this reservoir (Li et al. Reference Li, Yu, Lu, Cai, Bai and Xia2011). The most effective approach, however, is to prevent soil erosion in the source areas to avoid excessive sediment deposition in the TGR. Identifying the provenance of reservoir sediments is essential, therefore, to establish connections between sources and sinks, to focus on reducing sediment deposition by larger contributors, and to effectively solve the problem of sedimentation in the TGR.
Since the TGR commenced operation in 2003, many studies have examined the sediment provenance and weathering regimes in the TGR and in the Yangtze River (CWRC 2003–2017; Yang et al. Reference Yang, Zhang and Xu2007, Reference Yang, Milliman, Xu, Deng, Zhang and Luo2014; Chen et al. Reference Chen, Yan, Fu, Dou and Zhang2008; He et al. Reference He, Zheng, Huang, Jia and Li2013; Zhao et al. Reference Zhao, Zou, Gao, Wang, Li, Yao, Zhao and Xu2018). For example, CWRC (2003–2017) calculated the sediment inflow from upstream tributaries but excluded the sediments derived from the ungauged areas within the TGR watershed. The ungauged areas were taken into account by Yang et al. (Reference Yang, Zhang and Xu2007) and Chen et al. (Reference Chen, Yan, Fu, Dou and Zhang2008) but they assumed that the ratio of ungauged sediment inflow to gauged sediment inflow remained stable. The ungauged sediment discharge was estimated separately by Yang et al. (Reference Yang, Milliman, Xu, Deng, Zhang and Luo2014); their calculation was based on the assumption that larger sediment discharge from sources led to greater sediment deposition in the TGR, however, and did not consider the sediment transported downstream. These studies were calculated based on hydrological data only; other methods should also be applied to determine the sources accurately. Several studies have attempted to decipher the sediment provenance and weathering regime by clay mineralogy in the Yangtze River but have rarely focused on the TGR (He et al. Reference He, Zheng, Huang, Jia and Li2013; Zhao et al. Reference Zhao, Zou, Gao, Wang, Li, Yao, Zhao and Xu2018).
As important constituents of fine-grained sediments, clay minerals are widely distributed in fluvial and marine sediments (Gingele et al. Reference Gingele, De Deckker and Hillenbrand2001; Guyot et al. Reference Guyot, Jouanneau, Soares, Boaventura, Maillet and Lagane2007; Liu et al. Reference Liu, Colin, Huang, Phon Le, Tong, Chen and Trentesaux2007; Adriaens et al. Reference Adriaens, Zeelmaekers, Fettweis, Vanlierde, Vanlede, Stassen, Elsen, Środoń and Vandenberghe2018; Khan et al. Reference Khan, Liu, Liu, Seddique, Cao and Rahman2019). Clay mineral assemblages are sensitive to source-rock geology and chemical weathering and are considered to be effective indicators of the characteristics of the source areas; when they are entrained in a water mass, they can be transported over considerable distances from their source areas (He et al. Reference He, Zheng, Huang, Jia and Li2013; Liu et al. Reference Liu, Cao, Yin, Gao, Chen, Zhang and Yu2018; Zhao et al. Reference Zhao, Zou, Gao, Wang, Li, Yao, Zhao and Xu2018). Illite and chlorite usually reflect a dry-cold climate and/or low rainfall (Winkler et al. Reference Winkler, Wolf-Welling, Stattegger and Thiede2002; Pang et al. Reference Pang, Pan, Garzanti, Gao, Zhao and Chen2018). In contrast, kaolinite forms mainly due to pedogenesis, which is characterized by strong hydrolysis and complete removal of mobile cations (Vanderaveroet Reference Vanderaveroet2000; Pang et al. Reference Pang, Pan, Garzanti, Gao, Zhao and Chen2018). A high concentration of kaolinite implies a typically warm-humid climate. Montmorillonite forms in the early weathering process of unstable Fe-, Mg-, and Ca-rich minerals, which are contained in igneous or metamorphic rocks and distributed in temperate and cool climate zones characterized by moderate hydrolysis (Liu et al. Reference Liu, Colin, Li, Zhao, Tuo, Chen, Siringan, Liu, Huang, You and Huang2010; He et al. Reference He, Zheng, Huang, Jia and Li2013). In a hot and humid climate, montmorillonite is destroyed in the process of soil formation (Liu et al. Reference Liu, Colin, Li, Zhao, Tuo, Chen, Siringan, Liu, Huang, You and Huang2010; He et al. Reference He, Zheng, Huang, Jia and Li2013). Clay mineralogy can reveal the driving factors of weathering and clarify more recent trends in the climate and weathering conditions in the source areas (Wang et al. Reference Wang, Fan, Liu and Chang2016; Khan et al. Reference Khan, Liu, Liu, Seddique, Cao and Rahman2019). In summary, clay minerals can be regarded as useful tools for identifying sediment provenances, transport patterns, and weathering regimes in a basin. Major and trace-element compositions of fluvial sediments are controlled by weathering, lithologies, diagenesis, and sedimentary sorting and can also be used to identify geochemical processes and provenance of clastic materials (Yang et al. Reference Yang, Lim, Jung and Oh2004; Yang and Youn Reference Yang and Youn2007; Rao et al. Reference Rao, Tan, Chen, Ji, Chen, Pan and Zhang2015).
The work presented here is a comprehensive and coordinated study of mineralogical and geochemical data for sediments from the main stream and tributaries and soils from hillslopes in the TGR basin to understand: (1) the clay-mineral assemblages and geochemistry of sediments in the TGR; (2) the provenance of sediments during different seasons; and (3) the nature and extent of weathering in the catchment of the TGR. The present study provides a good example of studies in the field of clay science in China and adds to the significance of clay research (Zhou et al. Reference Zhou, Zhao, Wang, Chen and He2016).
Study Area
The TGR extends 663 km from Yichang to Chongqing along the main stream of the Yangtze River, with a total water surface area of ~1080 km2 (Fig. 1). The TGR was gradually impounded with phased increases in the water level to 135 m, 156 m, and 175 m in 2003, 2006, and 2010, respectively (Lu and Higgitt Reference Lu and Higgitt2001; Bao et al. Reference Bao, Gao and He2015). Since the impoundment behind the Three Gorges Dam (TGD), the water level has fluctuated between 145 m and 175 m, which interrupts the natural balance of sediment transport from the upper to lower reaches of the Yangtze River. The TGR operation is characterized by anti-seasonal water level changes; i.e. a high water level is maintained in the dry season, but a low water level is maintained in the flood season.

Fig. 1 Sketch map showing a the Yangtze River Basin, b Yangtze River major tributaries and main rock types, modified from Yang et al. (Reference Yang, Wang, Guo, Li and Cai2009), and c the Three Gorges Reservoir and sampling sites
The Three Gorges Dam intercepts the main channel of the Yangtze River at the outlet of its upper subbasin and controls a drainage area of ~1×106 km2 (Fig. 1) which produces major quantities of water and sediment (Yang et al. Reference Yang, Zhao and Belkin2002). The sediment deposition flux shows a decreasing trend in the TGR (CWRC 2003–2017; Huang et al. Reference Huang, Wang and Yang2019). Cascade dams such as Xiangjiaba, Xiluodu, Baihetan, and Wudongde will be located in the lower reaches of the Jinshajiang River; these dams are under construction or will be built on the main stream and tributaries of the upper Yangtze River. They may play an important role in reducing sediment transport and alleviating sediment deposition in the Three Gorges Reservoir. On the other hand, the continuous implementation of soil and water conservation and reforestation measures advocated by governments will help to reduce the suspended sediment flux in the Yangtze River (Tang et al. Reference Tang, Bao, He, Fu, Collins and Zhang2016).
The TGR basin has a monsoonal climate with annual mean air temperature and precipitation of 17–19°C and 1000–1200 mm, respectively. The winter is cold with an average temperature of 10°C and the summer is hot with an average temperature of 28–30°C (CNEMC 1997–2017). June to September is regarded as the flood season, while November to February is considered the dry season, and other months are known as the even season (Lou and Yin Reference Lou and Yin2016). A large fraction of the annual precipitation occurs from June to September (Xu et al. Reference Xu, Milliman and Xu2010). Soils are developed mainly based on weathered rocks in this basin (Bao et al. Reference Bao, Gao and He2015).
Tectonically, the TGR basin lies in the Yangtze Craton (Fig. 1c). This region is characterized by arrays of rolling hills and valleys. Clastic and carbonate rocks are distributed extensively with sporadic outcrops of magmatic and metamorphic rocks in this basin. Two different subregions are distinguished based on regional landforms and geology in this basin (Fig. 1). The upper-middle section is dominated by a hilly landscape and underlain by purple and red rocks composed of sandstone and mudstone. The lower section is a mountainous landscape with high mountains and deeply incised channels, and carbonate rocks comprise limestone and dolomite (Bao et al. Reference Bao, Gao and He2015).
The Yangtze River flows mostly among Mesozoic and Cenozoic rocks in the upper part of the Yangtze River Basin, which are underlain by Upper Paleozoic rocks. The upper basin consists of meta-igneous rocks and meta-sedimentary rocks, carbonate rocks, and igneous rocks, especially Himalayan intermediate-felsic igneous rocks. The middle-lower basins are composed mainly of Paleozoic marine and Quaternary fluviolacustrine sedimentary rocks, intermediate-felsic igneous rocks and ancient metamorphic rocks. Various tributary basins are composed of different source-rock types from various tectonic settings (Fig. 1).
Samples and Methods
Field Sampling
Surface sediment samples were collected from the top 5 cm of the sediment columns with a stratified sampler (DT-101B, Weifang, Shandong, China) onboard a ship in the Three Gorges Reservoir in July and December 2017 and in April 2018, except for the two samples from Wujiang and Longhe which were collected in April 2018 (Fig. 1). The hillslope soil samples in the TGR were collected during April 2018. All soil samples were obtained from the surface layer of 10 cm, and large dead leaves and stones were removed. These soils were mixed evenly and placed in polyethylene self-sealing bags for preservation. In total, the samples consisted of 53 surface sediment samples and ten soil samples. Each sample was treated as follows for mineralogical and chemical analyses. The <63 μm fraction was separated from the bulk sample by wet sieving and chosen as the study target, and this fraction was further treated at room temperature with 10% H2O2 (Hushi, Shanghai, China) for 48 h and 1 mol/L acetic acid (Hushi, Shanghai, China) for 24 h to remove organic matter, secondary carbonates, and adsorbed contaminants. Finally, the treated fraction was washed with ultrapure water, centrifuged, dried at 105°C in an oven (DUG-9240A, Shanghai, China), and powdered in an agate mortar. Physical and chemical pretreatments were performed to obtain provenance signals from the given grain-size residual fraction that were not affected by particle sorting and post-depositional processes (Rao et al. Reference Rao, Tan, Chen, Ji, Chen, Pan and Zhang2015).
Analytical Methods
First, organic matter and secondary carbonate were removed from bulk samples using 10% H2O2 and 0.1 mol/L HCl (Hushi, Shanghai, China). Then, the samples were fully dispersed by the addition of sodium hexametaphosphate (NaPO3)6 (Hushi, Shanghai, China). After this step, the grain-size measurements were performed using a laser diffraction particle analyzer (Mastersizer-2000, Cambridge, UK) at the Institute of Surficial Geochemistry, Nanjing University, China. The relative error was ~3% according to duplicate determinations.
Major elements were determined by X-ray fluorescence using an ARL 9800 spectrometer (Boston, Massachusetts, USA) at the Center of Modern Analysis, Nanjing University, China. The 0.6 g sample was mixed thoroughly with 6.6 g of the fluxing agents Li tetraborate (Kermel, Tianjin, China) and Li metaborate (Tianjin, China) (Li2B4O7/LiBO2 = 67/33) and 0.6 mL of 40 mg/mL of LiBr in a Pt-Au crucible (Tianjin, China). The mixture was fused using an automatic gas-fired CLAISSE burner system (M4, Panalytical, Beijing, China) to make a glass disc for XRF measurement. Work curves were established on the basis of the national geological standard samples (GBW07401–GBW07408, GBW07301–GBW07312, and GBW07103–GBW07108). Major-element concentrations were expressed as oxide concentrations from the output of instrumental measurements. The absolute errors were <±0.5% and <±0.3% for SiO2 and Al2O3, respectively. The relative errors were <5% for CaO, K2O, Fe2O3, and TiO2 and <10% for MgO, Na2O, P2O5, and MnO.
Trace element analysis was performed using an Agilent-7500a (Santa Clara, California, USA) inductively coupled plasma mass spectrometer (ICP-MS) at the University of Queensland (RIF-UQ), Australia. Each sample was dissolved in an acid mix (1:1) of distilled HF (Ajax Finechem, Melbourne, Victoria, Australia) and HNO3 (Ajax Finechem, Melbourne, Victoria, Australia) in a high-pressure jacket equipped with a Teflon beaker in an oven for 48 h to ensure complete digestion/dissolution. This procedure was repeated using larger amounts of acids for an additional 24 h. After digestion, the sample was evaporated to incipient dryness, refluxed with 6 N HNO3, and heated again to incipient dryness. The sample was then dissolved in 2 mL of 3 N HNO3 and diluted with Milli-Q water (18 MΩ cm) to a final dilution factor of 2000. The rock standards GSR-1, GSR-3, and AGV-2 were used to monitor the analytical accuracy and precision. The analytical accuracy, as indicated by the relative difference between the measured and recommended values, was better than 5%.
Scanning electron microscopy (SEM) analysis was performed using a Hitachi S-3400N (Tokyo, Honshu, Japan) instrument at the Center of Modern Analysis, Nanjing University, China. Representative bulk samples were prepared for SEM-EDX analysis by adhering the fresh broken surface of each rock sample using double-stick tape to an aluminum sample holder and coating the surface with a thin film of gold using a Giko ion coater (Hitachi E-1010, Tokyo, Honshu, Japan). The mineral composition of the <63 μm residual sample was determined qualitatively with an ARL-X’TRA (Boston, Massachusetts, USA) powder X-ray diffractometer with CuKα radiation, a voltage of 40 kV, a current of 40 mA, and continuous scans of 3–65°2θ at the Center of Modern Analysis, Nanjing University, China. Semi-quantitative estimates of relative mineral percentages were obtained from Cook et al. (Reference Cook, Johnson, Matti and Zemmels1975). The terms quartz, plagioclase, K-feldspar, and clay minerals were used here as general expressions for the respective mineral groups.
The clay fraction was separated from the bulk sample based on Stokes’s law, suspended in deionized water, and allowed to dry on glass slides to form oriented aggregates after removal of carbonate and organic matter. Then, the clay fraction was air dried, solvated for 12 h over a closed drying container with ethylene glycol (EG) in the bottom, covered by a lid coated with vacuum silicone grease in an oven at 60°C, then analyzed by XRD. This sample was heated to 550°C for 2 h and analyzed again by XRD. The XRD analyses were carried out using an X’TRA (Boston, Massachusetts, USA) X-ray diffractometer (Ni-filtered CuKα radiation, 40 kV voltage, and 40 mA current) with an error of <8% at the Center of Modern Analysis, Nanjing University, China. The clay slides were scanned from 3 to 35°2θ with a step size of 0.02°2θ. All clay minerals were identified and their peak areas obtained using the JADE software (Zhao et al. Reference Zhao, Zou, Gao, Wang, Li, Yao, Zhao and Xu2018). The terms montmorillonite, illite, kaolinite, and chlorite are used here as general expressions for the respective clay mineral groups. Semi-quantitative estimates of relative clay mineral percentages were obtained from measurements of XRD pattern peak areas, which were multiplied by the weighting factors from Biscaye (Reference Biscaye1965), namely, four times the illite peak area, two times the kaolinite + chlorite peak area, and one times the montmorillonite peak area, and then normalized to 100%. The relative proportions of kaolinite and chlorite were determined on the basis of the ratio of the 3.57/3.54 Å peak areas. Clay minerals were identified according to the position of the (001) series of basal reflections on the XRD patterns of air-dried, ethylene-glycolated, and heat-treated specimens. Illite was identified by the basal 10 Å peak; this basal spacing remained unaffected after the glycolation and heating treatments (Fig. 2). Kaolinite was recognized by its 7 Å peak, which disappeared after heating to 550°C. Montmorillonite exhibited a broad 12–15 Å reflection which increased to 17 Å after saturation with ethylene glycol. Chlorite was characterized by a first-order peak at 14 Å and a third-order peak at 4.7 Å, which did not expand when glycolated. After heating to 550°C, the 14 Å reflection was enhanced and shifted its position to 13.8 Å (Fig. 2).

Fig. 2 XRD traces of clay minerals in the TGR
Illite crystallinity was obtained from the half-height width of the 10 Å peak. Lower values represent greater crystallinity, characteristic of weak hydrolysis of continental source rocks and arid/cold climatic conditions (Chamley Reference Chamley1989; Ehrmann Reference Ehrmann1998). This index was also used as a potential tracer of source regions and transport paths. The illite chemistry index, which can mirror the weathering regime of the sediment provenance (Ehrmann Reference Ehrmann1998; Liu et al. Reference Liu, Trentesaux, Clemens, Colin, Wang, Huang and Boulay2003), refers to the ratio of the 5 and 10 Å peak areas. Ratios <0.5 represent Fe-Mg-rich illites (biotite micas), implying physical erosion; ratios >0.5 are found in Al-rich illites (muscovites), indicating strong hydrolysis (Gingele et al. Reference Gingele, De Deckker and Hillenbrand2001; Liu et al. Reference Liu, Tuo, Colin, Liu, Huang, Selvaraj, Chen, Zhao, Siringan, Boulay and Chen2008).
Data Analysis
One-way ANOVA was used to identify significant differences in clay mineral assemblages of the sediments during different seasons. The statistical analysis was performed with the IBM SPSS Statistics 22 software. All graphics were developed with the Origin 2016 software.
Results and Discussion
Grain Size, Mineral Composition, and Scanning Electron Microscopy
The main stream sediments were composed mainly of clay and silt with little sand (Supplementary Material Fig. S1). The clay (<4 μm) and silt (4–63 μm) fractions ranged from 17.8 to 39.4% and from 59.9 to 76.9%, respectively. The sand fraction (>63 μm) comprised <5% in most sediment samples. The main stream sediment had a regular grain-size variation with season (Fig. S1), i.e. the sediment was coarser during the even season, finer during the flood season, and intermediate during the dry season in the TGR.
Qualitative XRD analyses showed that common minerals were dominated by quartz, followed by clay minerals, plagioclase, and K-feldspar in the TGR sediments and hillslope soils (Fig. S2). The relative content of quartz was >50% in TGR main stream sediments throughout the three seasons and was >60% in TGR tributary sediments and hillslope soils (Table S1). The relative clay mineral content was ~20% in sediments and hillslope soils of the TGR. The plagioclase and K-feldspar contents were 13.3 and 8.4%, respectively, in sediments and hillslope soils of the TGR (Supplementary Material Table S1). Carbonate minerals were not detected by XRD, as a result of acid pretreatment.
The TGR sediments and hillslope soils were rich in clay minerals as revealed by SEM analysis (Fig. 3a). Fine-grained samples were coated with irregular flaky smectite and scaly illite (Gürel and Özcan Reference Gürel and Özcan2016; Gürel Reference Gürel2017). Books of kaolinite and leaf-shaped chlorite were also found in TGR sediments and soils (Fig. 3b,c). Moreover, honeycomb- or cotton-like mixed layers of illite-montmorillonite were scarcely present in the TGR sediments and soils (Fig. 3d).

Fig. 3 SEM images showing the sediment characteristics of the TGR
Geochemical Characteristics of TGR Sediments, Hillslope Soils, and Basement Rocks
Major and trace-element analyses of the TGR sediments and hillslope soils during the flood, dry, and even seasons (Table 2) revealed large concentrations of SiO2 and Al2O3 and small amounts of other elements. SiO2 and Al2O3 accounted for ~80% of the composition of individual samples, supporting the mineralogical results. The TGR main stream sediments showed larger amounts of Al2O3, Fe2O3, Cu, and Pb and smaller amounts of SiO2, Zr, and U than those of the tributary sediments and hillslope soils. Mean elemental concentrations did not differ greatly among the three seasons for the TGR sediments (Table 2). In addition, Wang et al. (Reference Wang, Li, Dong, Cui, Han, Zheng and Xu2018) reported that the major and trace element contents of basement rocks were lower than those of the sediments and soils in the TGR, especially Fe, Mg, Mn, P, Ti, V, Cr, Co, Ni, Cu, Zn, and Sc. The K, Na, Rb, Zr, Nb, and Hf contents in the basement rocks were slightly larger than those of the sediments and soils in the TGR, however (Fig. 4). The UCC-normalized patterns of the TGR sediments were roughly similar, especially in the major element section (Fig. 4). Relative to the UCC (Taylor and McLennan Reference Taylor and McLennan1985), TGR sediments and soil were enriched in Al2O3, Fe2O3, MgO, MnO, TiO2, Li, Cu, Zn, Nb, and Pb and partly in Ba, Sc, and some high field strength elements (Y, Zr, Nb, Pb, and Th) but depleted in other elements. While the basement rocks were distinctly depleted in Ca, Fe, Mg, Mn, P, and some metallic elements, other elements showed contents equivalent to those of the UCC (Fig. 4).

Fig. 4 UCC-normalized patterns of major and trace elements in sediments, hillslope soil, and basement rocks of the TGR. The data for basement rocks are from Wang et al. (Reference Wang, Li, Dong, Cui, Han, Zheng and Xu2018)
Clay-Mineral Compositions in the TGR Sediments
The clay-mineral assemblages of the main stream sediments were dominated by illite (65.7–80.6%), with an average of 74.7%. Kaolinite (8.9–16.9%) and chlorite (4.6–16.9%) were less abundant, with average contents of 12.5 and 9.5%, respectively. Montmorillonite (0.2–7.5%) was scarce, with a mean of 3.4% in the TGR main stream (Table 1). The illite chemical index ranged from 0.29 to 0.55 with a mean of 0.43, and the illite crystallinity values were in the range 0.44–0.60°Δ2θ with an average of 0.52°Δ2θ (Table 1).
Table 1 Clay-mineral assemblages of the main stream, tributary sediments and the hillslope soils of the Three Gorges Reservoir

The clay-mineral assemblages showed no significant differences among the three seasons (ANOVA, P>0.05) (Fig. 5). On average, the illite and kaolinite contents in the flood season (72.5 and 12.0%, respectively) were slightly smaller than those in the dry and even seasons (75.6 and 12.4%; 75.9 and 13.1%, respectively). The chlorite and montmorillonite contents in the flood season (average 10.9% and 4.7%, respectively) were slightly greater than those in the dry season and even season (averages 9.1 and 2.9%; 8.4 and 2.6%, respectively). The clay mineral assemblages did not show regular trends along the water-flow direction in the main stream sediments, however (Fig. 5).

Fig. 5 Clay-mineral compositions in the main stream sediments of the TGR
In addition to the main stream sediments, those of the TGR tributaries had clay-mineral assemblages that were dominated by illite (58.0–85.3%), with an average of 75.4% (Table 1). Kaolinite (4.2–17.9%) and chlorite (1.7–16.4%) were less abundant, with average contents of 10.6% and 9.5%, respectively (Table 1). Montmorillonite (0.4–11.2%) was scarce, with a mean of 4.6% in the TGR tributaries (Table 1). The clay-mineral contents in tributary sediments were equivalent to those in main stream sediments. The illite chemical index varied between 0.20 and 0.58 with a mean of 0.39 (Table 1); the illite crystallinity value ranged from 0.46–0.65°Δ2θ with an average of 0.52°Δ2θ (Table 1).
As shown in Fig. 6, the clay-mineral assemblages of each tributary river showed no obvious seasonal variations (ANOVA, P>0.05). On average, the montmorillonite and kaolinite contents in Xiangxihe were greatest, with means of 8.2 and 16.5%, respectively. The illite content in Meixihe was the largest, with a mean of 82.4%. The chlorite content in Changtanhe was greatest, with an average of 12.7%. The montmorillonite contents of the TGR tributaries were equivalent to those of upstream tributaries on the TGR except for Jialingjiang (Fig. 6). The kaolinite contents of the TGR tributaries were greater than those of Daduhe but less than those of Yalongjiang. The chlorite contents of the TGR tributaries were generally less than those of the upstream tributaries (He et al. Reference He, Zheng, Huang, Jia and Li2013).

Fig. 6 Clay-mineral assemblages in the sediments of upstream tributaries and TGR tributaries. The data for Jinshajiang, Yalongjiang, Daduhe, Minjiang, and Jialingjiang are from He et al. (Reference He, Zheng, Huang, Jia and Li2013)
The clay mineral assemblages of the TGR hillslope soils were dominated by illite (58.9–84.9%), with an average of 69.9%, less than those of main stream and tributary sediments on average (Table 1). Kaolinite (2.8–19.0%) and chlorite (8.3–25.6%) were less abundant, with average contents of 9.0 and 15.2%, respectively (Table 1). The kaolinite contents of the hillslope soils were close to those of main stream and tributary sediments, but the chlorite contents of the hillslope soils were much higher than those of main stream and tributary sediments (Table 1). Montmorillonite (2.1–11.5%) was scarce with a mean of 5.8% in the TGR hillslope soils, slightly higher than those of main stream and tributary sediments (Table 1). The illite chemical index was between 0.18 and 0.53 with a mean of 0.38, and the illite crystallinity value ranged over 0.33–0.72°Δ2θ with an average of 0.52°Δ2θ (Table 1). The illite chemical indices and the illite crystallinity values were similar in the main stream and tributary sediments and the hillslope soils (Table 1).
Clay minerals in the hillslope soils showed an obvious spatial trend from upstream to downstream of the TGR (Fig. 7). The kaolinite contents tended to increase, while the chlorite contents showed a decreasing trend, and the illite contents increased first and then decreased along the water-flow direction. The montmorillonite content showed no spatial variations, however (Fig. 7). The elevation decreased gradually, and the climate gradually became warm and humid along the water-flow direction of the TGR; thus, kaolinite developed more significantly (Bainbridge et al. Reference Bainbridge, Lewis, Smithers, Wilkinson, Douglas, Hillier and Brodie2016; Liu et al. Reference Liu, Cao, Yin, Gao, Chen, Zhang and Yu2018).

Fig. 7 Clay-mineral assemblages of the hillslope soils in the TGR
Provenance of the TGR Sediments under Regular Dam Operation
Erosion rates were calculated to range between 0.18 and 0.6 mm/y for the upstream tributaries of the Yangtze River according to the depositional fluxes and concentrations of river sediments (Chappell et al. Reference Chappell, Zheng and Fifield2006; Godard et al. Reference Godard, Lavé, Carcaillet, Cattin, Bourlès and Zhu2010; Kong et al. Reference Kong, Zheng and Fu2011; Vezzoli et al. Reference Vezzoli, Garzanti, Limonta, Andò and Yang2016). The annual soil loss in the TGR was also estimated to be 1.57⋅108 t, of which 4⋅107 t entered the Yangtze River at the rate of 700 t km−2 y−1 (Liu et al. Reference Liu, Xiao, Liu, Zhang and Zhang2016a). In addition, the TGR tributaries produced large amounts of sediment inflows every year (Tang et al. Reference Tang, Bao, He, Fu, Collins and Zhang2016). In summary, the principal sediments supplied to the Three Gorges Reservoir could be divided into three groups with different sources based on their spatial scales: distal source (upstream tributaries such as Jinshajiang), regional source (TGR tributaries such as Xiangxihe), and proximal source (hillslope soil erosion in the TGR).
Among the distal sources, the clay-mineral compositions of Jinshajiang and Minjiang were closer to that of the TGR than those of the other three rivers (Yalongjiang, Daduhe, and Jialingjiang), especially Jinshajiang, which plotted in the center of the TGR field, while Minjiang was closer to the samples from the dry and even seasons (Fig. 8). All tributaries were generally close in clay-mineral compositions to the main stream sediments in the TGR. Values from the samples of the hillslope soils were scattered, and the hillslope soils contributed more to the sediments in the TGR during the flood season but less to the sediments in the other two seasons (Fig. 8). This was because frequent large rainfall events caused serious erosion of hillslope soils in the flood season. Large amounts of sediment were deposited in the TGR due to heavy rainfall (Bao et al. Reference Bao, Gao and He2015, Reference Bao, He, Wen, Gao, Tang, Yan and Long2018). In summary, the distal sources, including Jinshajiang and Minjiang (in the dry and even seasons), and the TGR tributaries contributed much sediment to the TGR main stream, while the TGR hillslope soils made a greater contribution during the flood season than in the other two seasons.

Fig. 8 Ternary diagram of the major clay-mineral groups: illite + chlorite, kaolinite, and montmorillonite in the TGR and rivers worldwide
Some major elements (Al, Fe, and Mg), other transition metals (e.g. Sc, Cr, and V), and high field strength elements are considered stable in a hypergene environment, and their ratios might represent the whole-rock composition of the source (Taylor and McLennan Reference Taylor and McLennan1985). Therefore, pairs of these elemental ratios have been used widely to trace the provenance of sediments (Yang et al. Reference Yang, Lim, Jung and Oh2004; Rao et al. Reference Rao, Tan, Chen, Ji, Chen, Pan and Zhang2015). The geochemical data of the distal source were summarized by He et al. (Reference He, Zheng, Clift, Tada, Wu and Luo2015). From the elemental triangular diagrams (Fig. S3), geochemical similarities and differences between the TGR sediments and their potential sources could be observed more clearly. In general, TGR main stream sediments were constrained by each potential source, which indicated that TGR sediments were supplied by all three sources. Seasonally, the distal source contributed more to the TGR main stream sediments in the flood season than in the other two seasons. Spatially, the ternary diagrams of trace elements indicated that the distal source was the most important contributor, which was consistent with the results from clay minerals, while the major element ternary diagrams indicated that proximal and regional sources contributed more. Yang and Youn (Reference Yang and Youn2007) suggested that the fine fraction could not ultimately eliminate the grain-size effect, which influenced the provenance results from major elements. Nevertheless, the geochemical data supported the conclusions regarding clay minerals.
The annual sediment discharge of each source was summarized based on the hydrological data (Table 2) (CWRC 2003–2017; Yang et al. Reference Yang, Milliman, Xu, Deng, Zhang and Luo2014; Liu et al. Reference Liu, Xiao, Liu, Zhang and Zhang2016a). The annual sediment discharge of the Jinsha River was greatest among the distal sources, and the annual sediment discharges from regional and proximal sources were also considerable (Table S2). The annual sediment discharge data from hydrological stations further supported the mineralogical results.
Table 2 Average concentration of major and trace elements of sediments and hillslope soil in the Three Gorges Reservoir

Implications for the Weathering Regime of River Sediments
Clay minerals in river sediments are good indicators of the weathering intensity across the watershed, and their compositions are controlled mainly by lithology, climate, structure, terrain environment, vegetation, and soil development (Mao et al. Reference Mao, Chen, Yuan, Yang, Balsam and Ji2010; Bi et al. Reference Bi, Yang, Li, Guo, Wang, Liu and Yin2015; Wang et al. Reference Wang, Fan, Liu and Chang2016). The clay-mineral compositions of river sediments varied widely in different regions (Fig. 8 and Table S3). Rivers such as the Yangtze River (including the TGR area) that originated from plateaus or tall mountains were dominated by illite (Table S3). These observations implied that illite was a residue produced by the physical decomposition of micas in metamorphic rocks or of feldspar to sericite (Garzanti et al. Reference Garzanti, Padoan, Setti, López-Galindo and Villa2014). Compared with the Yangtze River as a whole, however, the TGR contained sediments that showed mainly significant illite crystallinity and low illite chemical index, which indicated that the sediments were subjected to significant physical weathering and to weak hydrolysis of rocks (Fig. 9). These processes occurred mainly because the upstream lithologies in the Yangtze River, as the main source of sediments in the TGR, were subjected mainly to physical weathering in cold-arid climates (Zhao et al. Reference Zhao, Zou, Gao, Wang, Li, Yao, Zhao and Xu2018). The TGR sediments showed seasonal variations. The chemical index of TGR sediments was higher in the flood season than in the dry and even seasons (Fig. 9). Moreover, the chemical index of sediments in the flood season was >0.4, which meant that the sediment contained Al-rich illite and had experienced intense hydrolysis, but the sediments in the other two seasons had Fe- and Mg-rich illite, resulting from physical weathering (Chamley Reference Chamley1989; Liu et al. Reference Liu, Tuo, Colin, Liu, Huang, Selvaraj, Chen, Zhao, Siringan, Boulay and Chen2008; He et al. Reference He, Zheng, Huang, Jia and Li2013). Throughout the whole Yangtze River Basin, the disparities in illite indices between the northern and southern tributaries were remarkable (Fig. 9). Northern tributaries of the Yangtze River had greater illite and smaller kaolinite abundances than those of southern tributaries, which reflected the differences in their climates and weathering environments. The southern tributaries had a relatively warmer and more humid climate together with stable morphology and low relief (He et al. Reference He, Zheng, Huang, Jia and Li2013).

Fig. 9 Correlation between the illite chemistry index and the illite crystallinity of the sediments in the TGR and other rivers
In sharp contrast, the montmorillonite contents in rivers (Luzon and Ganges Rivers) from the tropics and subtropics were very large (Fig. 8 and Table S3). This occurred because the formation of montmorillonite requires a warm climate with the rainy season separated from the dry season with strong evaporation (Velde Reference Velde1995; Liu et al. Reference Liu, Colin, Li, Zhao, Tuo, Chen, Siringan, Liu, Huang, You and Huang2010; He et al. Reference He, Zheng, Huang, Jia and Li2013). The long-lasting and intense hydrolysis due to the low-relief and stable morphology caused greater kaolinite abundances in the Pearl, Mekong, Zhejiang, and Fujian Rivers (Liu et al. Reference Liu, Colin, Huang, Phon Le, Tong, Chen and Trentesaux2007, Reference Liu, Zhao, Colin, Stattegger, Wiesner, Huh, Zhang, Li, Sompongchaiyakul, You, Huang, Liu, Siringan, Le, Sathiamurthy, Hantoro, Liu, Tuo, Zhao, Zhou, He, Wang, Bunsomboonsakul and Li2016b; Khan et al. Reference Khan, Liu, Liu, Seddique, Cao and Rahman2019). Meanwhile, the Taiwanese rivers, the Red river, the Mekong River, the Pearl River, and the Bengal Basin Rivers are situated at lower latitudes than the Yangtze River (including the TGR area). They are located at similar latitudes and are influenced by typhoons and monsoon cycles with warm and humid weather systems, which cause the illite indices of these river sediments to fall within a relatively small range (Fig. 9). More intense chemical weathering in the Pearl River, however, was attested by higher illite indices than those in the other rivers (Liu et al. Reference Liu, Colin, Huang, Phon Le, Tong, Chen and Trentesaux2007). The Bengal Basin rivers sediments were formed by physical weathering processes because of the small illite chemical index values (<0.4) (Khan et al. Reference Khan, Liu, Liu, Seddique, Cao and Rahman2019). The sediments in Taiwanese rivers had much lower FWHM values than other Asian rivers, which reflected strong physical erosion (Wang et al. Reference Wang, Fan, Liu and Chang2016).
Some scholars have suggested that weathering intensity may be affected by climate and geographical location (Wang et al. Reference Wang, Fan, Liu and Chang2016; Khan et al. Reference Khan, Liu, Liu, Seddique, Cao and Rahman2019). The relationships between the meteorological parameters (precipitation and temperature), latitude, and illite crystallinity in the Asian rivers were explored. As shown in Fig. 10, the influences of latitude and temperature on the illite crystallinity were small, while precipitation had a significant effect on illite crystallinity; i.e. the greater the annual precipitation, the stronger the chemical weathering effect. In summary, precipitation was the dominant factor affecting the weathering intensity.
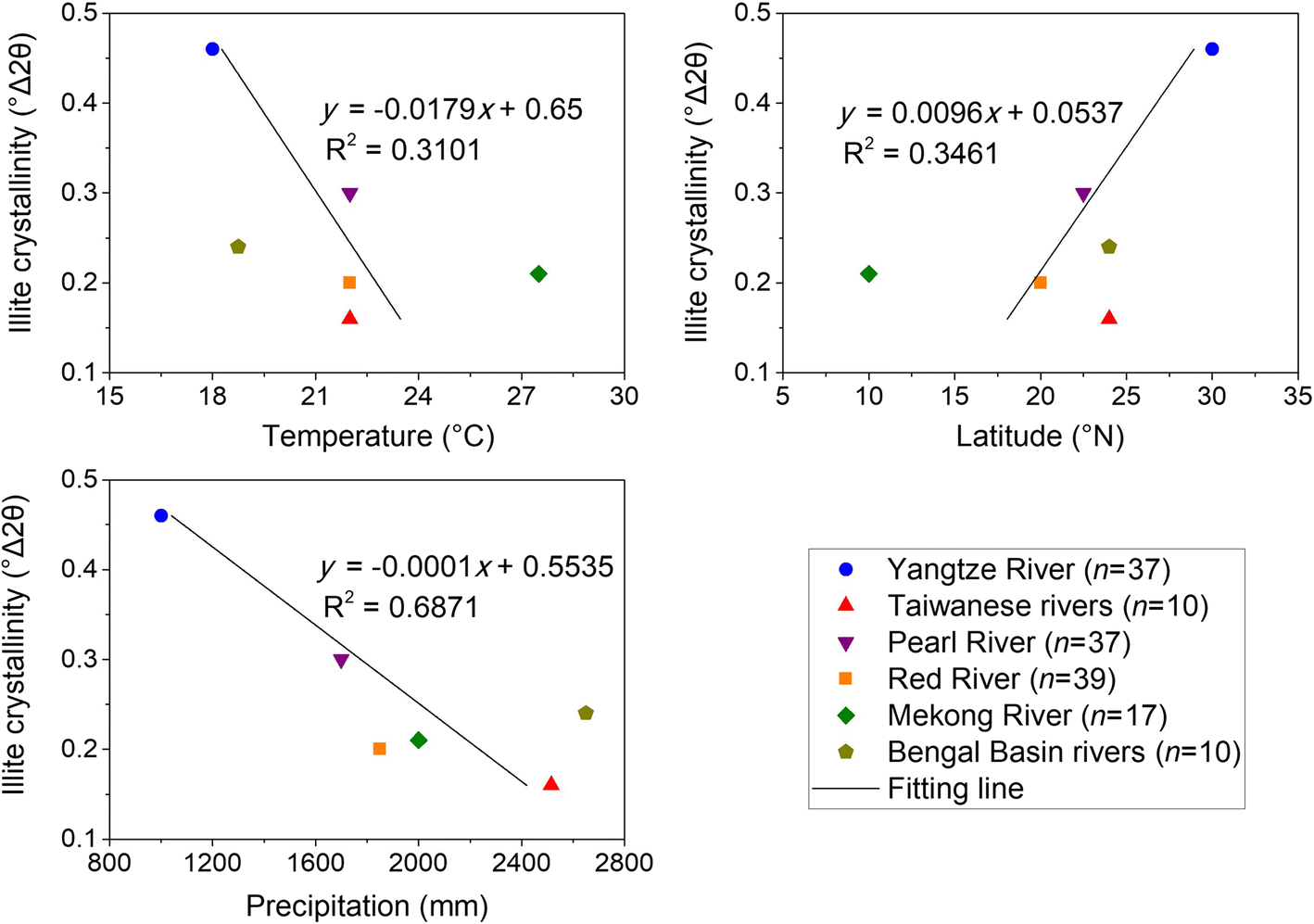
Fig. 10 Diagrams of illite crystallinity vs. temperature, latitude, and precipitation in the TGR sediments. The data f temperature, latitude, and precipitation were from He et al. (Reference He, Zheng, Huang, Jia and Li2013) for the Yangtze River, from Li et al. (Reference Li, Shi, Kao, Te Chen, Liu, Fang, Lü, Zou, Liu and Qiao2012) for the Taiwanese rivers, from Liu et al. (Reference Liu, Colin, Huang, Phon Le, Tong, Chen and Trentesaux2007) for the Pearl, Red, and Mekong Rivers, and from Khan et al. (Reference Khan, Liu, Liu, Seddique, Cao and Rahman2019) for the Bengal Basin rivers
Conclusions
The clay mineralogy and geochemistry of main stream and tributary sediments and hillslope soils collected from the Three Gorges Reservoir (TGR) Basin, China, were investigated to identify sediment provenances and weathering regimes.
The results indicated that common minerals were dominated by quartz, followed by clay minerals, plagioclase, and K-feldspar in the TGR sediments and hillslope soils. Illite, kaolinite, chlorite, and montmorillonite were identified using SEM. Clay-mineral compositions throughout the TGR were dominated by illite, with less kaolinite and chlorite, and scarce montmorillonite. The amounts of illite and kaolinite in the flood season were slightly smaller than those in the dry and even seasons, but the amounts of chlorite and montmorillonite in the flood season were slightly greater than in the other two seasons. Large amounts of SiO2 and Al2O3 and small amounts of other elements were distinctive features of TGR sediments and soils, while the mean elemental concentrations did not differ greatly among the three seasons for the TGR sediments.
Three major sources of sediments were distinguished in the TGR. Based on the analysis of mineralogical, geochemical, and hydrological data, the distal sources, including Jinshajiang and Minjiang (in the dry and even seasons), contributed much sediment to the TGR. The regional source, which includes all the TGR tributaries, was also a main contributor during the flood, dry, and even seasons, and the proximal source, hillslope soils, contributed more in the flood season than in the other two seasons.
Compared to the whole Yangtze River, the TGR contained sediments that had high illite crystallinity and a low illite chemical index, reflecting strong physical weathering and weak hydrolysis of rocks. The chemical indices of sediments during the flood season in the TGR were mostly >0.4, which meant that the sediments consisted of Al-rich illite and had experienced intense hydrolysis, but the sediments during the other two seasons consisted of Fe- and Mg-rich illite resulting from physical weathering. In addition, chemical weathering was affected mainly by precipitation, while temperature and latitude had less influence.
Supplementary Information
The online version contains supplementary material available at https://doi.org/10.1007/s42860-020-00106-5.
Acknowledgments
This study was supported financially by the National Key Research and Development Program of China (Grant No. 2016YFC0401703), the Fundamental Research Funds for the Central Universities (B200203111), the Natural Science Foundation of Jiangsu Province (Grant No. BK20191304), the Fundamental Research Funds for the Central Universities (Grant No. 2019B45414), the Postgraduate Research & Practice Innovation Program of Jiangsu Province (KYCX20_0490), and China Scholarship Council (CSC). Dr Tianning Li and Xiaofan Ma are acknowledged for help with the experiments.
Funding
Funding sources are as stated in the Acknowledgments.
Compliance with Ethical Statements
Conflict of Interest
The authors declare that they have no conflict of interest.